- 1Hainan Yazhou Bay Seed Laboratory, Sanya Nanfan Research Institute of Hainan University, Sanya, China
- 2Collaborative Innovation Center of Nanfan and High-Efficiency Tropical Agriculture, Hainan University, Haikou, China
- 3School of Integrative Plant Science, Section of Plant Biology and the L.H. Bailey Hortorium, Cornell University, Ithaca, NY, United States
- 4BTI Computational Biology Center, Boyce Thompson Institute, Ithaca, NY, United States
- 5Zhai Mingguo Academician Work Station, Sanya University, Sanya, China
- 6Hainan Shengda Modern Agriculture Development Co., Ltd., Qionghai, China
Arecaceae is a species-rich clade of Arecales, while also being regarded as a morphologically diverse angiosperm family with numerous species having significant economic, medicinal, and ornamental value. Although in-depth studies focused on the chloroplast structure of Arecaceae, as well as inferring phylogenetic relationships using gene fragments, have been reported in recent years, a comprehensive analysis of the chloroplast structure of Arecaceae is still needed. Here we perform a comprehensive analysis of the structural features of the chloroplast genome of Arecaceae, compare the variability of gene sequences, infer phylogenetic relationships, estimate species divergence times, and reconstruct ancestral morphological traits. In this study, 74 chloroplast genomes of Arecaceae were obtained, covering five subfamilies. The results show that all chloroplast genomes possess a typical tetrad structure ranging in size between 153,806-160,122 bp, with a total of 130-137 genes, including 76-82 protein-coding genes, 29-32 tRNA genes, and 4 rRNA genes. Additionally, the total GC content was between 36.9-37.7%. Analysis of the SC/IR boundary indicated that the IR region underwent expansion or contraction. Phylogenetic relationships indicate that all five subfamilies in Arecaceae are monophyletic and that Ceroxyloideae and Arecoideae are sister groups (BS/PP = 100/1). The results of molecular dating indicate that the age of the crown group of Arecaceae is likely to be 96.60 [84.90-107.60] Ma, while the age of the stem group is 102.40 [93.44-111.17] Ma. Reconstruction of ancestral traits indicate that the ancestral characteristics of the family include monoecious plants, one seed, six stamens, and a smooth pericarp.
Introduction
Arecaceae is a family belonging to the Arecales, ranking fifth among monocot families in terms of species richness. According to APG IV (Angiosperm Phylogeny Group et al., 2016), Arecaceae is divided into five subfamilies (Calamoideae, Nypoideae, Coryphoideae, Ceroxyloideae, and Arecoideae), with 181 genera and approximately 2600 species currently recognized (Dransfield et al., 2005; Dransfield et al., 2008; Baker and Dransfield, 2016). Members of Arecaceae are widely distributed in tropical and subtropical regions all over the world (Govaerts and Dransfield, 2005; Dransfield et al., 2008; Baker et al., 2009; Trias-Blasi et al., 2015), with only a few species extending into temperate regions. Arecoideae, the largest and most diverse subfamily in Arecaceae, includes approximately 60% of the genera and 50% of the species in Arecaceae (Dransfield et al., 2008). The family originated in North America, with subsequent diversification of most tribes having occurred in the Americas (Comer et al., 2016).
Arecaceae is also one of the most morphologically diverse angiosperm groups with a variety of morphological characteristics (Dransfield et al., 2008). Most typical forms are non-branching arbors, some are shrubs, and very few species are lianas or without aboveground stems. The surface of the plant stem is smooth, rough, or spiny, and covered with remnants of old petiole bases or leaf scars; the inflorescence is usually large and multi-branched or surrounded by spathes; the shape and size of the fruits are diverse (Figure 1). Thomas and De Franceschi (2013) noted that each of the five subfamilies and their tribes have distinct distinguishing features. Arecaceae is a large economic family comparable to Gramineae, including food crops, oil crops, sugar crops, fruits, and other economic crops with great value, such as Cocos nucifera, Phoenix dactylifera, and Elaeis guineensis (Dransfield et al., 2008; Fadini et al., 2009). Numerous species have been widely cultivated as ornamentals and are indispensable species to courtyards and road landscapes (Wang et al., 2014; Tang et al., 2018). There are also some edible and medicinal plants, such as Cocos nucifera in Arecoideae, which have important medicinal value such as a therapeutic effect on diabetes (Joseph et al., 2019), while Butia eriospatha is not only used for ornamental purposes but also has edible fruits (de Souza Magnabosco et al., 2020). Plants of Arecaceae also have great anthropogenic uses, such as leaves, fruits, seeds, and fibers serving as a basic public resource for traditional communities and which have even been exploited commercially on a large scale (Johnson, 2010; Kissling et al., 2019).
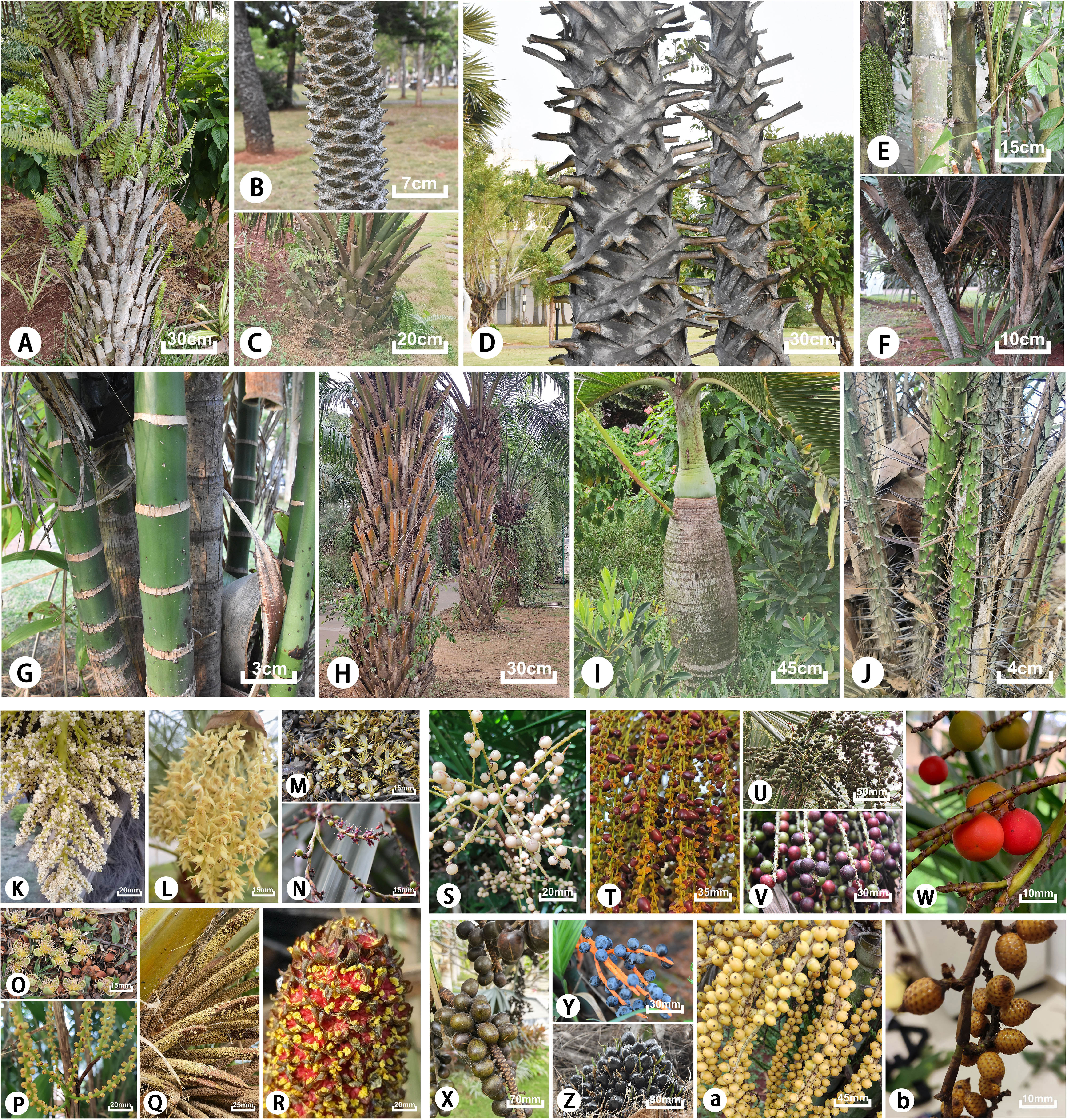
Figure 1 Typical morphological characteristics of tribes of the subfamilies in Arecacea. Coryphoideae: (A) Copernicia alba; (B, L, T) Phoenix roebelenii; (C, U) Sabal minor; (D) Borassus flabellifer; (E, M, V) Caryota mitis; (F, N, W) Chuniophoenix hainanensis; (K) Trachycarpus fortunei; (S) Lanonia dasyantha; (X) Bismarckia nobilis; Arecoideae: (G) Areca triandra; (H, Q, Z) Elaeis guineensis; (I) Hyophorbe lagenicaulis; (O) Wodyetia bifurcata; (P, Y) Chamaedorea elegans; (a) Ptychosperma macarthurii; Calamoideae: (J, R) Salacca zalacca; (b) Calamus rhabdocladus.
Members of Arecaceae have nearly every possible combination of hermaphrodite or unisexual flowers observed among the numerous angiosperm families, and it is this feature that makes the family a great system for studying the evolution of plant sexuality. However, in most evolutionary studies, the sampling has been relatively small with only a few chloroplast fragments used. Therefore, the phylogenetic relationship of Arecaceae at the subfamily level are still not clear, with support of some nodes being quite low and the phylogenetic position of some taxa remaining controversial. Wang (2007) inferred the phylogenetic relationships among 18 genera of native Chinese Arecaceae using sequences of the matK intron and ribosomal transcribed spacer (ITS). The results showed that the ITS sequences of some Arecaceae plants were drastically different within species, with some conspecific plants being more different than those of non-conspecific plants. Asmussen et al. (2000) constructed a phylogenetic relationship for 65 Arecaceae species by combining the rps16 intron and trnL-trnF region and found that the Nypoideae + Calamoideae clade formed a sister group to the rest of the family with moderate support. Comer et al. (2015) studied the phylogenetic relationships of Arecoideae using plastids obtained by next-generation sequencing and showed that Arecoideae was monophyletic with the tribe Chamaedoreaeae diverging first. That study identified three major evolutionary clades in the group: the Reinhardtieae, Roystoneeae, Cocoseae (RRC) clade, the Podococceae, Oranieae, Sclerospermeae (POS) clade, and the core arecoids clade (Areceae, Euterpeae, Geonomateae, Leopoldinieae, Manicarieae, Pelagodoxeae). Baker et al. (2009; 2011) also supported Arecoideae as monophyletic, but the tribe Iriarteeae was found to be the earliest divergent lineage. The nuclear genome phylogeny inferred by Comer et al. (2016) also supports the tribe Iriarteeae as the earliest divergent lineage. However, in the study by Pichardo-Marcano et al. (2019) using three nuclear and one chloroplast DNA markers to infer a Bayesian maximum clade credibility tree, the results showed that the tribes Chamaedoreaeae and Iriarteeae are sister groups to each other. Bacon et al. (2016) generated data from five plastid (ndhA, petD-rpoA, psbK-trnS, trnG and trnD-trnT) and six nuclear (AG1, CISPs 4 and 5, PRK, RPB2 and WRKY21) loci to infer the phylogenetic relationships of tribe Iriarteeae using Bayesian analysis, showing that all genera were inferred to be monophyletic and the affinities between genera were strongly to moderately supported. Based on the four plastid intergenic spacers (psbA-trnH, psbZ-trnfM, atpI-atpH, and rps3-rpl16), Faye et al. (2014) performed a phylogenetic analysis on the species-level subtribe Ancistrophyllinae in the Calamoideae using maximum parsimony, maximum likelihood and Bayesian analysis. The results showed that the Ancistrophyllinae and genera within the subtribe are strongly supported as monophyletic. Barrett et al. (2019) analyzed the phylogenetic relationships, biogeography and evolution of Brahea in the Coryphoideae, the ML and Bayesian analysis of whole aligned plastomes strongly supported Brahea as monophyletic. The majority of previous studies are based on the analysis of plastids at the tribe/genera level, while rarely analyzing the phylogenetic relationship at the subfamily level (Hahn, 2002b; Faye et al., 2014; Heyduk et al., 2016; Barrett et al., 2019).
Although some plastomes of Arecaceae have been reported, most studies have focused on the genus or species level, and no comprehensive plastome analysis of Arecaceae has been carried out. Therefore, based on extensive sampling around the world, this study uses chloroplast genome data to address the following three scientific goals: 1. Analyze the chloroplast genome structure of Arecaceae; 2. Establish robust phylogenetic relationships of Arecaceae at the subfamily level; 3. Estimate the divergence time of each subfamily through and combine with existing traits to reconstruct ancestral traits of Arecaceae species.
Materials and methods
Taxon sampling, DNA extraction and sequencing
In this study, leaf material of 24 species of Arecaceae, covering three subfamilies and 22 genera, were collected, and the leaves were stored in silica gel. Total genomic DNA was extracted from silica-dried leaf material using a modified cetyltrimethyl ammonium bromide (CTAB) method (Doyle and Doyle, 1987). Quality and quantity of the DNA was assessed using 1% agarose gel electrophoresis and an ultra-micro spectrophotometer (ultra-micro nucleic acid analyzer). Before library construction and whole genome sequencing of DNA, we quantified and analyzed each sample using an Agilent 2100 BioAnalyzer (Davis, California, USA), and selected DNA samples with a total content of at least ≥0.8 ug. We constructed paired-end sequencing libraries with an insert size of 300-500 bp and performed sequencing using the BGISEQ-500 platform at the Beijing Genome Research Institute (BGI; Shenzhen, China). Raw reads were filtered and trimmed using SOAPfilter_v2.2 with the following standard parameters: (1) screening for low quality base reads (>10% Ns and/or >40% low quality bases); (2) screening for reads generated by PCR duplication; (3) trimming of adapter sequences. All newly sequenced raw reads have been submitted to the Sequence Read Archive (SRA) under BioProject PRJNA748537 (see Table 1 for details such as collection location, GenBank number, etc.). The collection of the 24 newly sequenced samples was approved by Hainan University (Hainan, China) and complied with local policy requirements. In addition, we downloaded 50 species of Arecaceae (covering five subfamilies) and three species of Asparagaceae as outgroups from the National Center for Biotechnology Information (NCBI), the details are shown in Table 2. Thus, a total of 74 Arecaceae chloroplast genomes representing five subfamilies and 54 genera were used for analyses.
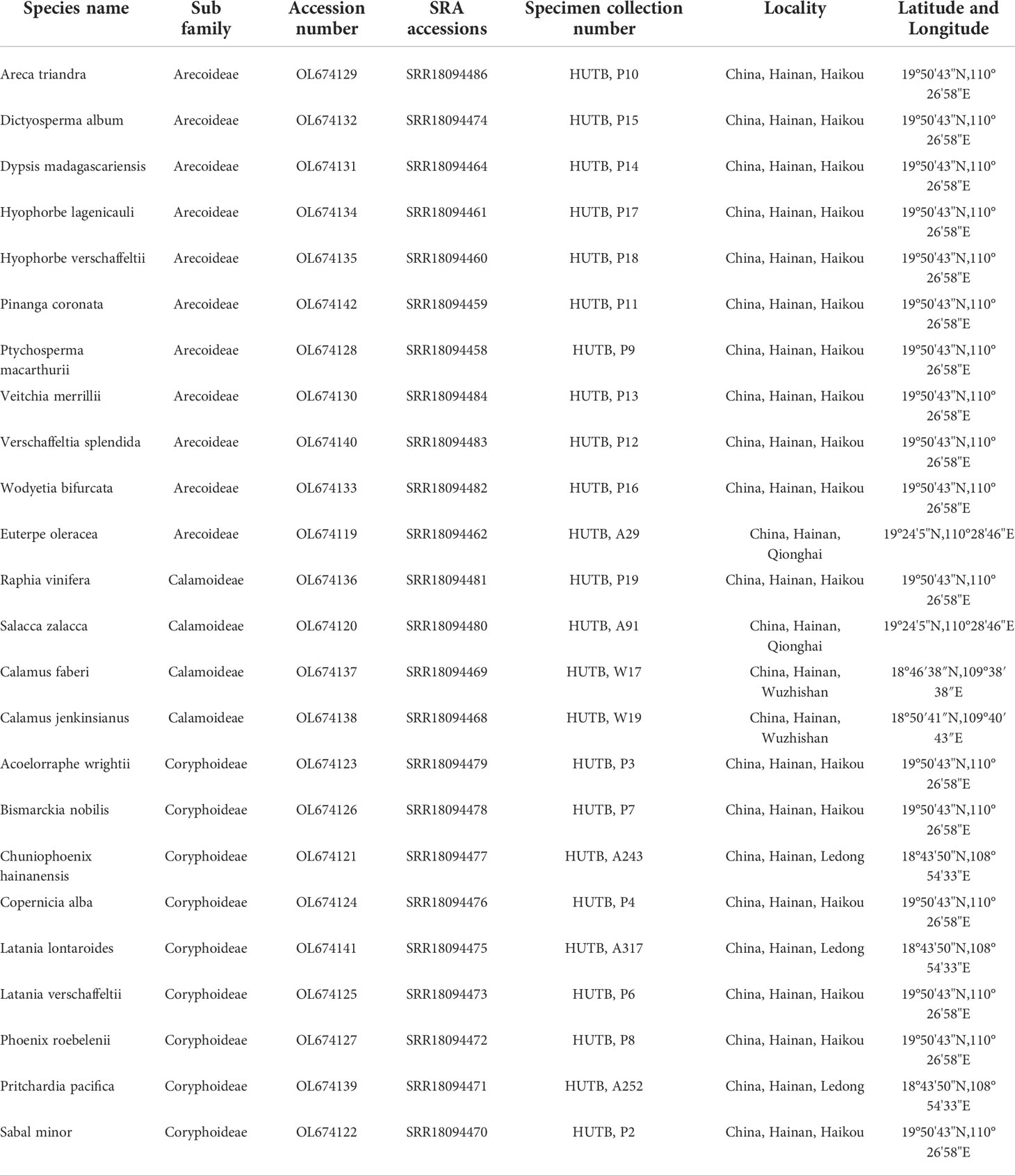
Table 1 GenBank number, SRA number and collection location information of 24 newly sequenced chloroplast genomes in Arecaceae.
Plastome assembly and annotation
We used GetOrganelle v1.7.5.0 (Jin et al., 2020) to assemble the chloroplast genomes from the clean reads of each species, with default parameters (see the online manual available at https://github.com/Kinggerm/GetOrganelle). The newly assembled slimmed assembly graph (FASTG) and selected target assembly graph (GFA) were visualized by Bandage v0.8.1 (Wick et al., 2015) to assess the completeness of the final assembly graph. The Mauve v1.1.3 (Darling et al., 2004) alignment was used to check the collinearity of genomic sequences before annotation of the sequences. The plastome sequences were initially annotated with Geneious Prime v2021.2.2 (Kearse et al., 2012), using close relatives as reference sequences, with further manual editing of the start codons, stop codons, and intron/exon boundaries. tRNAscan-SE1.21 was used to verify tRNA genes (Schattner et al., 2005). Plastome maps were drawn with OrganellarGenomeDRAW (OGDRAW) v1.3.1 (see https://chlorobox.mpimp-golm.mpg.de/OGDraw.html) (Lohse et al., 2013). All newly annotated plastomes sequences have been submitted to NCBI (see Table 1 for GenBank numbers).
Plastome comparative analysis and sequence differences
Based on the whole chloroplast genome phylogeny in this study (Figure 5), we selected 30 representative species (samples were selected by genus, including 21 newly sequenced samples and nine samples downloaded from NCBI, covering five subfamilies) for comparative analysis. Plastome comparisons across the 30 representative species were performed in Shuffle-LAGAN mode on the mVISTA program (see genome.lbl.gov/vista/index.shtml) (Frazer et al., 2004), with Acrocomia aculeata (NC_037084.1) as the annotation reference. We used Unipro UGENE v38.1 (Rose et al., 2019) to confirm the IR region. Photoshop was used to draw the IR/SC boundary map of the thirty chloroplast genomes of Arecaceae.
Phylogenetic analysis
We inferred phylogenetic relationships using 77 species of Arecaceae with three species of Asparagaceae serving as outgroups: Ophiopogon bodinieri (NC_051508.1), Ophiopogon jaburan (NC_049870.1) and Dracaena fragrans (NC_054234.1). Plastome sequences were aligned with MAFFT v7.313 (Katoh and Standley, 2013) and aligned columns with more than 90% missing data were removed using Phyutility (Smith and Dunn, 2008). For ML and BI inference, we generated two datasets, one of the protein-coding sequences (CDSs) and the other with complete plastome sequences. Additionally, we analyzed other data subsets for phylogenetic relationships (i.e. Non-coding regions, LSC, SSC and IRb regions, whole plastome sequence minus one Inverted Repeat copy sequence (No-IRA)). Maximum likelihood analyses were conducted using IQ-TREE v1.6.8 (Nguyen et al., 2015), while searching for the best partition scheme (Lanfear et al., 2012) followed by ML tree inference and 1000 ultrafast bootstrap replicates (Hoang et al., 2018). Bayesian analyses were performed using MrBayes v3.2.7 (Ronquist et al., 2012). We used Akaike Information Criterion (AIC) in JMODELTEST v2.1.7 (Santorum et al., 2014) to determine the best-fitting model of molecular evolution was GTR+I+G (Table 3). Each Markov chain Monte Carlo (MCMC) run was conducted for 50 million generations sampling every 1000 generations. The first 25% of trees were discarded as burn-in, and the remaining trees were used to construct a consensus tree to estimate the posterior probabilities (PPs). We used Tracer v1.7.2 (Rambaut et al., 2018) to evaluate convergence and effective sample size (ESS > 200). All phylogenetic trees were visually analyzed using FigTree v1.4.4 (http://tree.bio.ed.ac.uk/software/figtree).
Divergence time estimation
We performed a dated phylogenetic analysis using BEAST v2.6.6 (Drummond et al., 2012) to investigate the historical biogeography of Arecaceae. The BEAST analysis used a Yule speciation prior and an uncorrelated log normal (UCLN) relaxed clock to estimate the divergence time. The best performing model of molecular evolution was selected as the GTR+I+G model according to AIC selected by MrModelTest v2.4 (Posada, 2008). The age of the crown node of Arecaceae was calibrated using fossil calibration points and secondary calibration points. According to Khan et al. (2020) the well-preserved petrified palm stem fossils, Palmoxylon ceroxyloides, dating to 66-65 Ma from the Deccan Traps, was identified as the oldest stems of Ceroxyloideae in the fossil record. Therefore, we set a log-normal prior for the stem of Ceroxyloideae clade with a fossil crown age of 65.0 Ma. Based on the rich Arecaceae fruit fossil record of Matsunaga and Smith (2021), they suggest that some fossils were suitable as node calibrations. Here, we used two of these fossils as node calibrations. We used a log-normal distribution to set the crown age of Trachycarpeae to 62.0 Ma and the crown group age of Areceae to 47.0 Ma. Based on the phylogeny estimated by Li et al. (2019), the crown group age of Arecales was constrained to 96.2 Ma, setting a secondary calibration point of the crown age of Arecaceae+Asparagaceae branch to 96.2 Ma using a normal prior with a mean=96.2 Ma and stdev=5. The MCMC chain length set at 9 x108 generations sampling every 1000 generations. We used Tracer v1.7.2 (Rambaut et al., 2018) to evaluate convergence and effective sample size (ESS > 200), while discarding the first 10% of trees. LogCombiner v2.6.6 (Drummond et al., 2012) was used to eliminate burn-in trees and merge tree files from nine runs, and the first 25% of trees were discarded as burn-in. TreeAnnotator v2.6.6 (Drummond et al., 2012) was used to generate a maximum clade credibility (MCC) tree. The final inferred tree was visually analyzed using FigTree v1.4.4 (http://tree.bio.ed.ac.uk/software/figtree/) showing the mean divergence time estimates with 95% maximum posterior density (HPD) intervals.
Morphological evolution analysis of Arecaceae
This study analyzed the historical reconstruction of ancestral morphological characters of key traits in Arecaceae based on the topology of the whole chloroplast genome for ML analysis, using the “Trace character history” option in Mesquite v3.51, and the Markov k-state reference model (Maddison, 2008). The data for the morphological characteristics of Arecaceae were mainly obtained from our field observations of the samples and the literature (Moore and Uhl, 1982; Dransfield et al., 2008; Horn et al., 2009; Matsunaga and Smith, 2021). The morphological traits are shown in Table 5. Associated codes for species-specific traits that were scored include: A) Number of stamens: (0) 3; (1) 6; (2) 10-40; (3) 40+; B) Number of seeds: (0) 1; (1) 1-2; (2) 1-3; (3) 3; (4) 4-10; C) Pericarp type: (0) Smooth; (1) Rough; (2) Spiny; (3) Imbricate scales; D) Plant type: (0) Monoecious; (1) Dioecious.
Results
Structural features of chloroplast genomes
In this study, we investigated 74 chloroplast genomes in Arecaceae, covering five subfamilies: Arecoideae (24 species in five tribes), Calamoideae (11 species in three tribes), Ceroxyloideae (two species in two tribes), Coryphoideae (36 species in eight tribes) and Nypoideae (one species in one tribe). The results show that all 74 chloroplast genomes display the typical tetrad structure of angiosperms: a LSC region (82,900–87,639 bp), a SSC region (13,768–18,380 bp), and a pair of IR regions (26,007–28,228 bp) (Figure 2; Table 2). The length of the 74 plastomes range from 153,806 to 160,122 bp, with a size difference of 6,316 bp. The differences in the LSC, SSC and IR regions span 4,739 bp, 4,612 bp and 2,221 bp, respectively. The plastome length of the 24 species of Arecoideae range from 154,048 bp (Butia eriospatha, NC_058633.1) to 159,237 bp (Euterpe oleracea, OL674119), while the plastomes of the 11 species of Calamoideae range from 154,031 bp (Eremospatha macrocarpa, NC_029964.1) to 158,029 bp (Calamus jenkinsianus, OL674138) in length. The plastome length of the two species of Ceroxyloideae are 157,829 bp (Pseudophoenix vinifera, NC_020364.1) and 159,075 bp (Phytelephas aequatorialis, NC_029957.1). The length of the 36 species of Coryphoideae range from 153,806 bp (Chuniophoenix nana, NC_029966.1) to 160,122 bp (Latania lontaroides, OL674141), and the length of the one species of Nypoideae is 158,391 bp (Nypa fruticans, NC_029958.1). All Arecaceae plastomes encode a total of 130-137 genes, of which 112-117 genes (76-82 unique protein-coding genes, 29-32 tRNA genes, and 4 rRNA genes) are located in the single copy regions and 18-20 genes are duplicated in the IR regions. The total GC content of the plastomes are highly similar, ranging from 36.9-37.7%, and the average GC content of the plastome was 37.3%, while the GC content in the LSC, SSC and IR regions are 34.8-35.9%, 31.0-31.6%, and 42.3-42.8%, respectively (Tables 2, 4). In addition, multiple genome alignments were performed on 74 Arecaceae plants to determine whether Arecaceae plastomes were rearranged. The Mauve alignment results are detailed in the attachment (Figure S1).
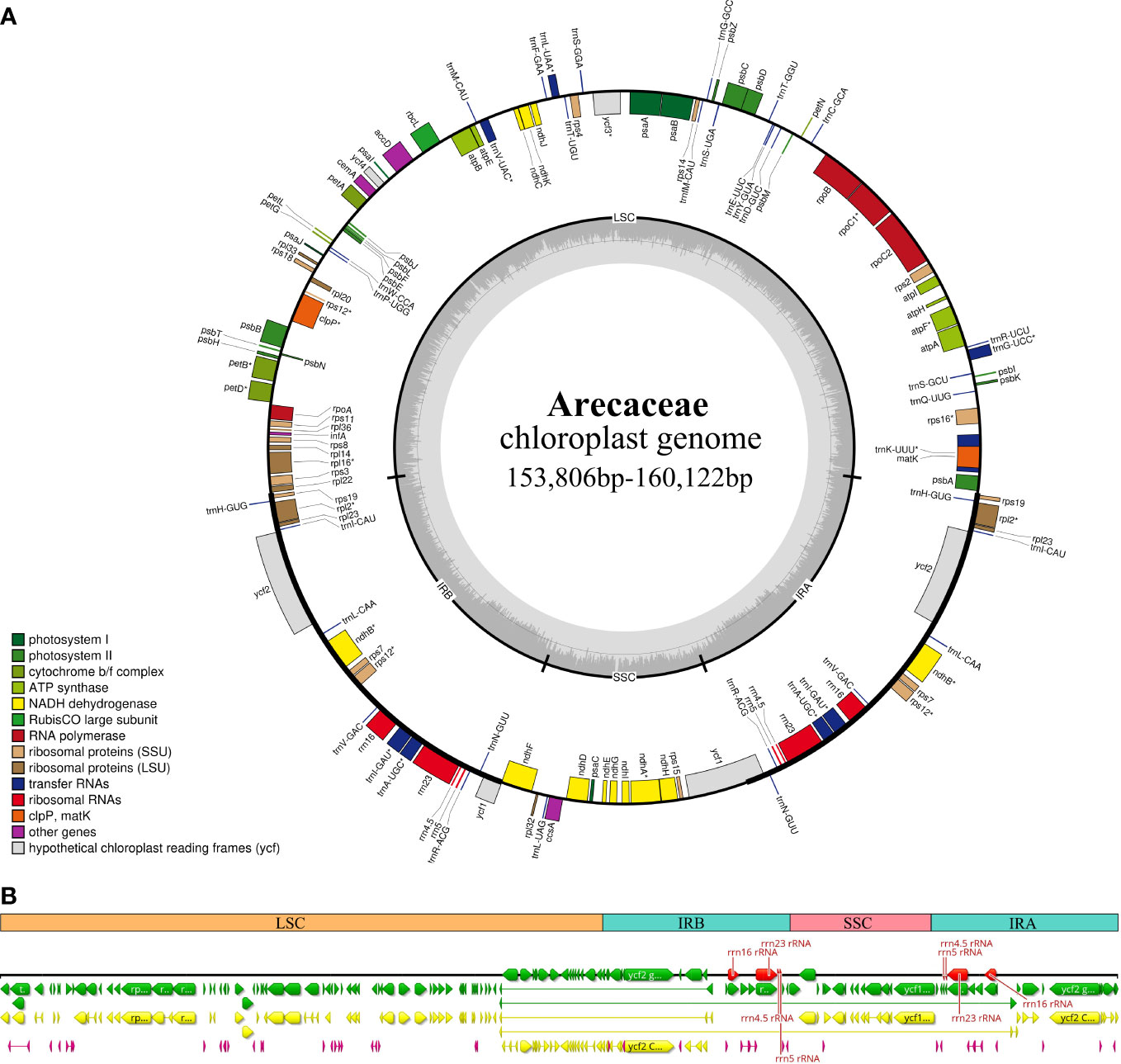
Figure 2 Chloroplast genome map of Arecaceae. (A) Circular form. Genes inside the outer circles are transcribed clockwise and those outside the circles are transcribed counterclockwise. The gray variation area in the inner circle indicates the GC content of the chloroplast genome. Different colors indicate different functional genes. (B) Linear form. Different colors indicate different functional genes, green indicates Genes, yellow indicates Protein Coding Genes, red indicates rRNA genes, and purple indicates tRNA genes.
Comparative genomic analysis and SC/IR boundary comparisons
In this study, we used mVISTA to analyze the sequence differences of 30 representative chloroplast genomes in Arecaceae, using Acrocomia aculeata as a reference. The alignments show that the plastomes of the 30 Arecaceae species have few differences (Figure 3). The sequence identity of the coding regions are higher than that of non-coding regions, and the sequence identity of the IR region is higher than that of the SC region.
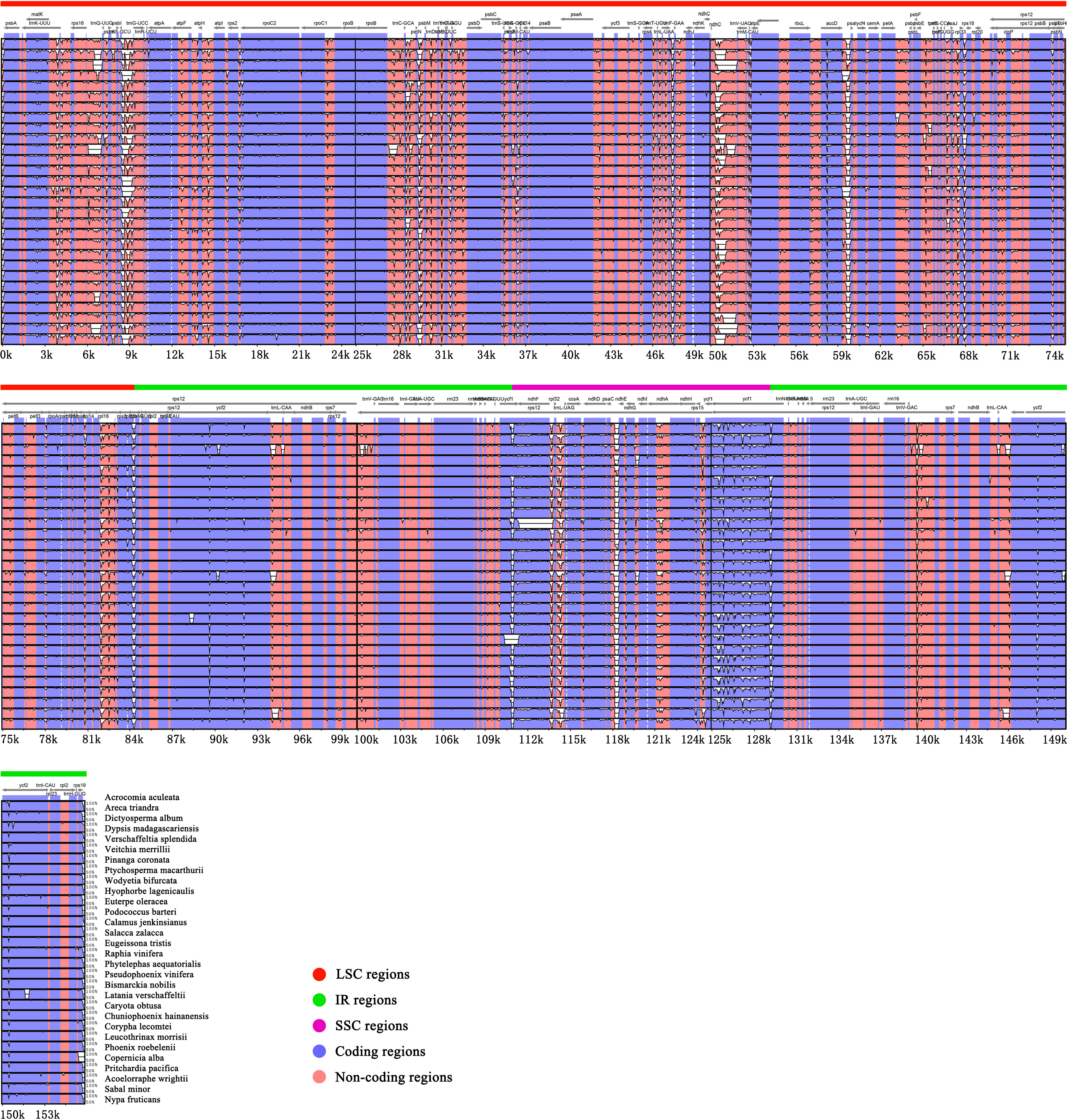
Figure 3 Comparative visualization of chloroplast genome sequence differences in Arecaceae. The protein coding and non-coding regions correspond to blue and red. The x-axis uses Acrocomia aculeata as reference sequence and y-axis indicates the percentage of sequence identity (50%-100%).
The results show the differences between the SC and IR boundary regions are structurally similar across the 30 species, and the boundary genes between SC/IR regions are stable. The same genes had the same relative position at the junction of SC/IR regions, with a few exceptions (Figure 4). At the LSC/IRb junction, rpl22 and rps19 were detected in 28 chloroplast genomes (rpl22 gene is located in the LSC and rps19 gene is located in the IRb), while the LSC/IRb junction of Dictyosperma album was detected as rps19 and rpl2 (rps19 gene is located in the LSC and rpl2 is located in the IRb), and Pseudophoenix vinifera showed that rps19 straddles the LSC/IR boundary, with a length of 48 bp in the IRb. Except for Eugeissona tristis, the gene detected at the IRb/SSC boundary was rpl32 (the gene was located in the SSC), and ndhF was detected across the IRb/SSC boundary in other species. ycf1 was detected at the SSC/IRa boundary in all 30 species. For one species, Dictyosperma album, rpl2 and psbA (rpl2 is located in the IRa) were detected at the IRa/LSC boundary, while rps19 and psbA were detected in all other species.
Phylogenetic results
Based on 74 whole plastomes, the topology generated by ML and BI phylogenetic inference were consistent with full support for each node [ML bootstrap (BS) = 100%, Bayesian posterior probabilities (PP) = 1] (Figure 5). At the subfamily level, the phylogenetic tree fully showed that the five subfamilies were all monophyletic, and the relationships between genera had high support. In the phylogenetic trees, Ceroxyloideae and Arecoideae are sister groups, and Coryphoideae was confirmed as the sister group of Ceroxyloideae + Arecoideae (BS/PP = 100/1). Nypoideae was identified as the sister of [Coryphoideae+ [Ceroxyloideae +Arecoideae]] with strong support, while Calamoideae was identified as the sister to all other Arecaceae with full support (BS/PP = 100/1). The phylogenetic trees (ML/BI) inferred using the LSC, No-IRa, protein-coding sequence (CDS) and non-protein-coding sequence (Non-CDS) (Figures S2, S5, S6, S7), showed relationships at the subfamily level consistent with those using whole plastomes. However, using just the SSC region, a separate clade of Coryphoideae and the clade of [Nypoideae+[Coryphoideae+[Ceroxyloideae+Arecoideae]]] formed a sister relationship, and Coryphoideae was not monophyletic (Figure S3). In the phylogenetic tree reconstructed with the IRb region, Ceroxyloideae and Coryphoideae were found to be sisters, while Arecoideae was the sister group of Ceroxyloideae+Coryphoideae and was not monophyletic with high support (Figure S4).
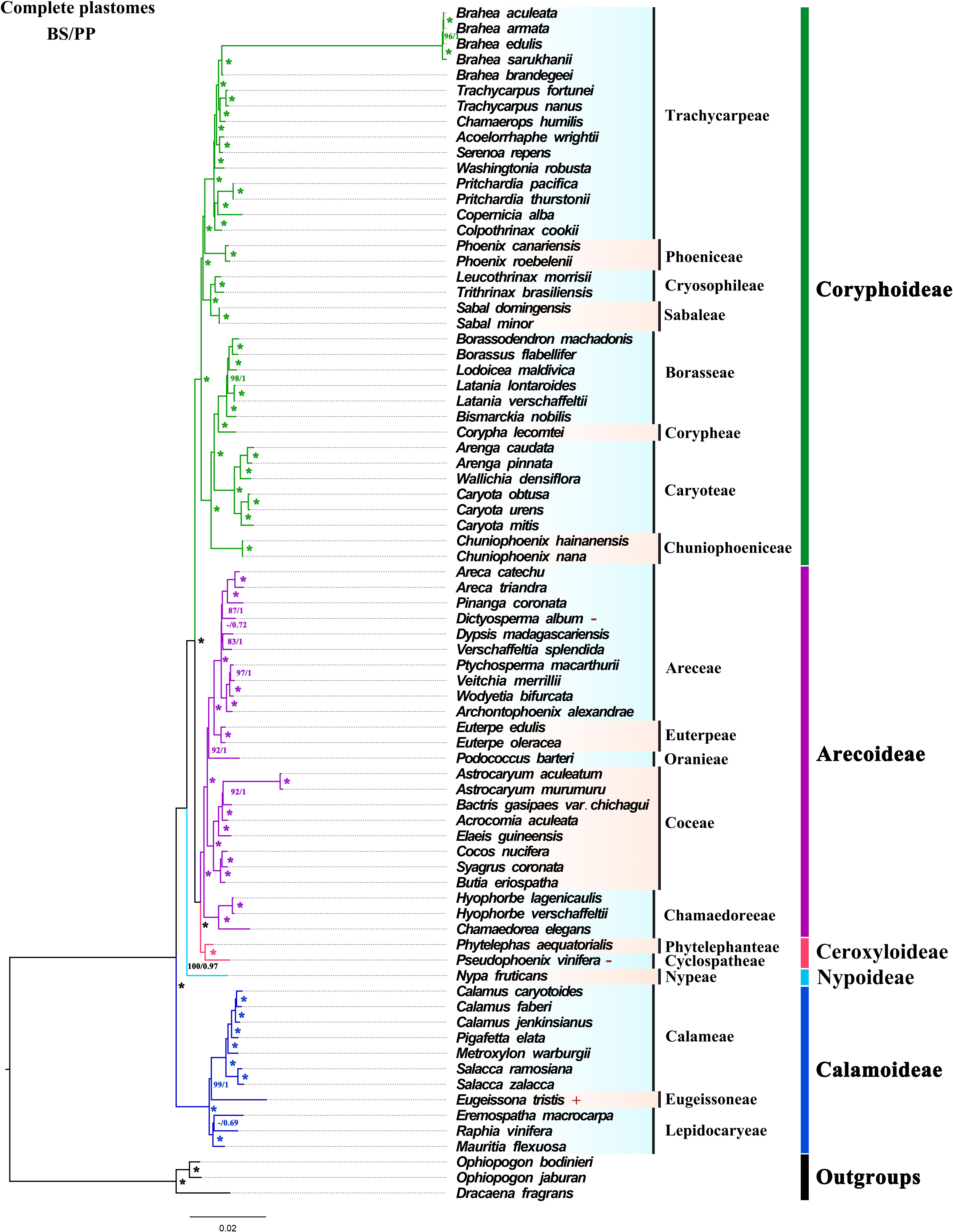
Figure 5 ML and BI trees were constructed based on the whole chloroplast genome dataset. “*” indicate support values of 100%/1.0, numbers near the nodes indicate 60% and 0.6 or more support obtained from the analysis, and “-” is used when both support values are less than 60% or 0.6. Different colors represent different clades of subfamilies. The “+” presented to the right of the species indicates that the species expands at the IR/SC boundary, and “-” indicates that the species contracts at the IR/SC boundary.
Divergence time estimation of Arecaceae
We estimated the divergence time using the 74 whole plastomes of Arecaceae using BEAST (Figure 6). The crown group age of Arecaceae was 96.60 Ma (95% HPD = 84.90–107.60 Ma), and the stem age was 102.40 Ma (95% HPD = 93.44–111.17 Ma), which corresponds to the Early Cretaceous. The clades of Nypoideae and [[Ceroxyloideae+Arecoideae]+Coryphoideae] (crown group age: 84.47 Ma, 95% HPD = 75.57–93.68 Ma) diverged 89.37 Ma during the Late Cretaceous. Approximately 84.47 Ma (95% HPD = 75.57–93.68 Ma) the Ceroxyloideae and Arecoideae clade diverged from the Coryphoideae, and the crown group of Coryphoideae was dated to 77.50 Ma (95% HPD = 68.61–86.98 Ma). The crown group of Ceroxyloideae (crown group age: 66.32 Ma, 95% HPD = 65.03–68.99 Ma) and Arecoideae (crown group age: 69.52 Ma, 95% HPD = 59.49–79.85 Ma) diverged 76.15 Ma (95% HPD = 67.53–85.42 Ma). In addition, the Calamoideae crown group diverged 53.39 Ma (95% HPD = 16.99–87.13 Ma) during the Eocene.
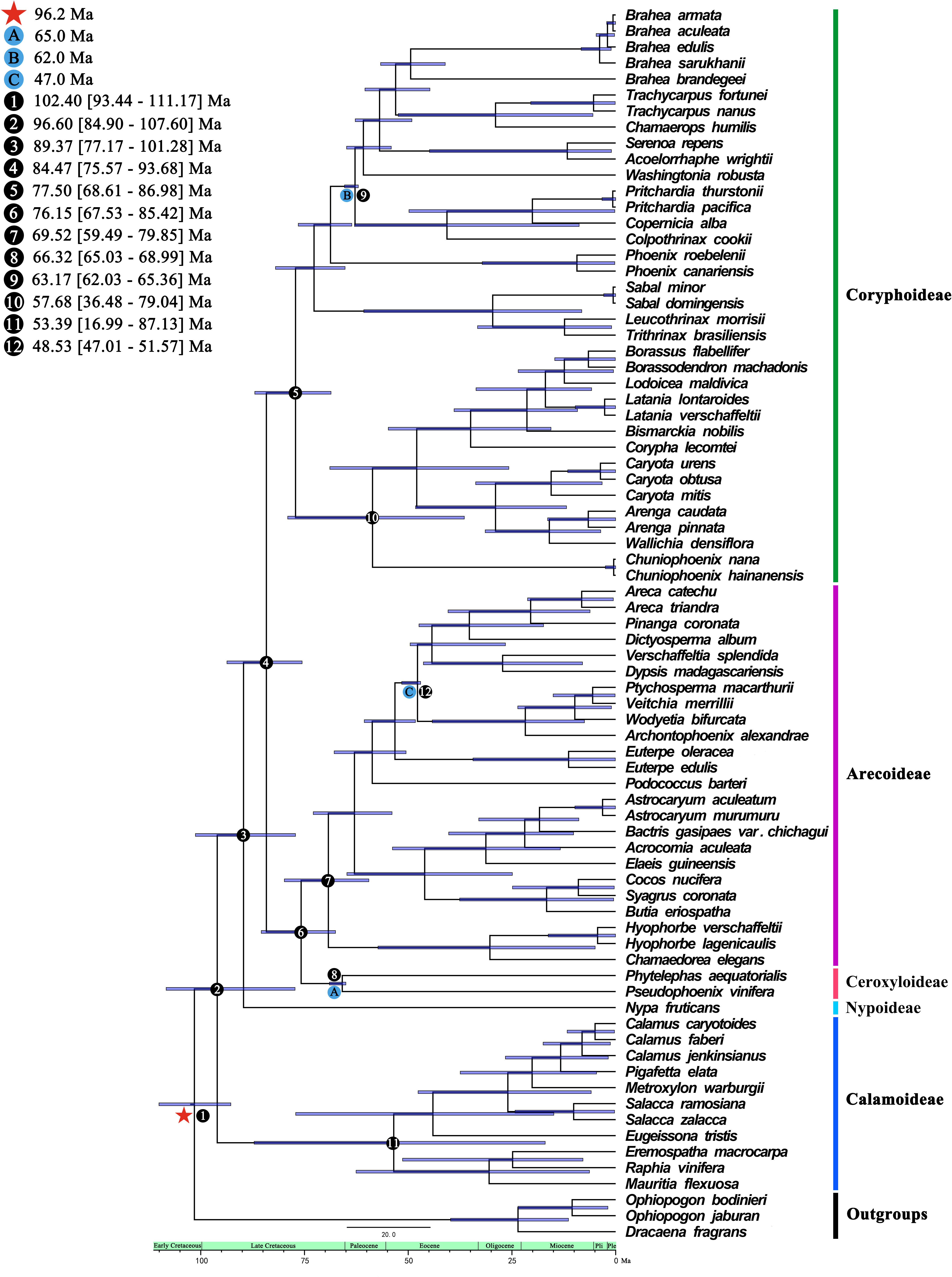
Figure 6 Species divergence times based on whole chloroplast genome datasets analyzed from BEAST. A, B, and C are calibration points, respectively, and star shape are secondary calibration points. Numbers 1-12 represent the estimated mean divergence times of the main divergence events and 95% highest posterior densities.
Ancestral trait reconstruction
The evolutionary analysis of species morphology in Arecaceae shows that some traits evolved via convergent evolution. For example, the trait of pericarp type reflects this phenomenon (Figure 7). For this trait, we infer that the ancestral fruit pericarp trait of Arecaceae plants was smooth. The fruit pericarp of species in Calamoideae were all imbricate scales, whereas species in Ceroxyloideae have evolved to have a rough fruit pericarp, and plants of Phytelephas in the tribe Phytelephanteae appear to have a rough acute-warty pericarp. In Arecoideae, only species of Astrocaryum and Bactris in the tribe Coceae have evolved a spiny pericarp, while all other species retain the ancestral trait. In addition, in the Coryphoideae all species retain the original smooth pericarp of the ancestral form. In terms of plant types, the ancestral plant type of Arecaceae was monoecious, which then evolved into a dioecious plant (Figure 7). Except for the species of tribe Eugeissoneae and Eremospatha and Raphia in tribe Lepidocaryeae, which still maintain the original ancestral form in Calamoideae, other species have evolved into dioecious plants. The species of Phytelephas in Ceroxyloideae and species of Chamaedorea of tribe Chamaedoreaee in Arecoideae have also evolved into dioecious plants. Similar diversity changes have occurred in Coryphoideae, for example, the plants of tribe Borasseae and Phoenixe have evolved dioecy, while in the tribe Trachycarpeae, most species still retain the ancestral form during the evolutionary process.
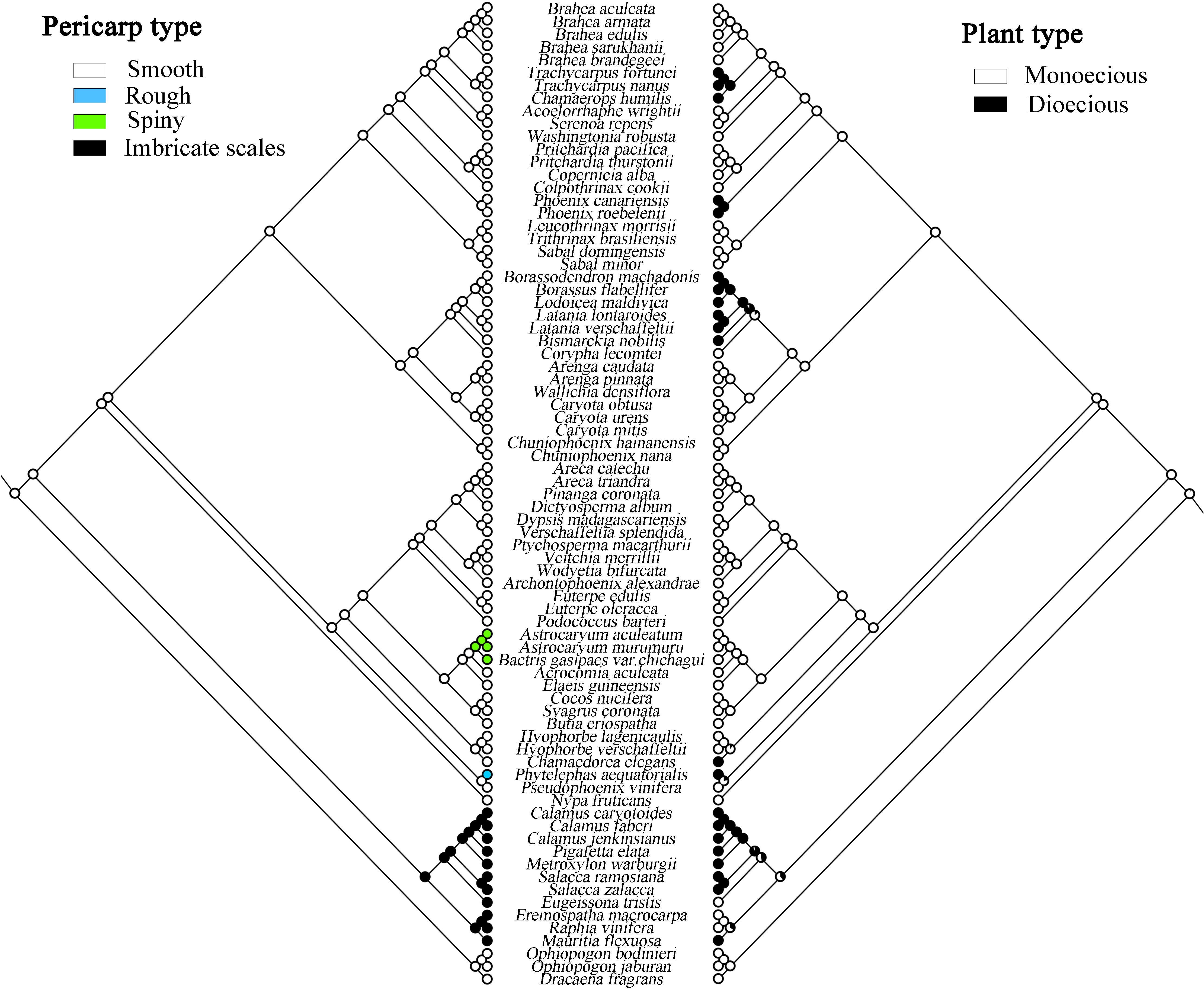
Figure 7 Maximum likelihood analysis of morphological traits evolution in Arecaceae based on the chloroplast data set. Left, Pericarp type; Right, Plant type.
The present study infers that species having one seed is the ancestral trait in Arecaceae, while some plants evolved to have more seeds (Figure 8), such as in Ceroxyloideae, Pseudophoenix of the tribe Cyclospatheae with one to three seeds and even four to 10 seeds in Phytelephas of the tribe Phytelephanteae. The number of seeds of species in Calamoideae and Coryphoideae show high diversity, for example Salacca in the tribe Calameae of Calamoideae independently evolved three seeds, species of Eremospatha in the tribe Lepidocaryeae evolved one to three seeds, while in Coryphoideae some species of the tribe Borasseae evolved independently one to three or three seeds, and in the tribe Caryoteae, seeds ranged from one to two or one to three seeds. In Arecoideae, plants of Butia in the tribe Coceae and plants of Podococcus in the tribe Oranieae also evolved one to three seeds independently. Otherwise, the number of seeds in the other Arecaceae species has largely maintained the ancestral form. We also found that the evolution of the number of stamens was complex and infer that having six stamens was the ancestral state of Arecaceae, with multiple instances of evolving a greater number of stamens (Figure 8) (Table 5). In the Calamoideae, only plants of Eugeissona in the tribe Eugeissoneae and plants of Raphia in the tribe Lepidocaryeae have changed in the number of stamens. Species of Phytelephas of the tribe Phytelephanteae in Ceroxyloideae have a large disparity in the number of stamens, ranging from dozens to hundreds. In the Arecoideae only species of the tribe Areceae have evolved diversity, with numbers varying by dozens, while Areca triandra have undergone degeneration in stamen number, with numbers reduced to three, and the same degeneration occurring in Nypa fruticans of Nypoideae, with stamen numbers also reduced to three. The remaining tribes in Coryphoideae retain their ancestral traits, in addition to the evolution of stamens in species of two tribes, the Borasseae and the Caryoteae. Overall, most plants have undergone long-term evolution from their ancestral forms.
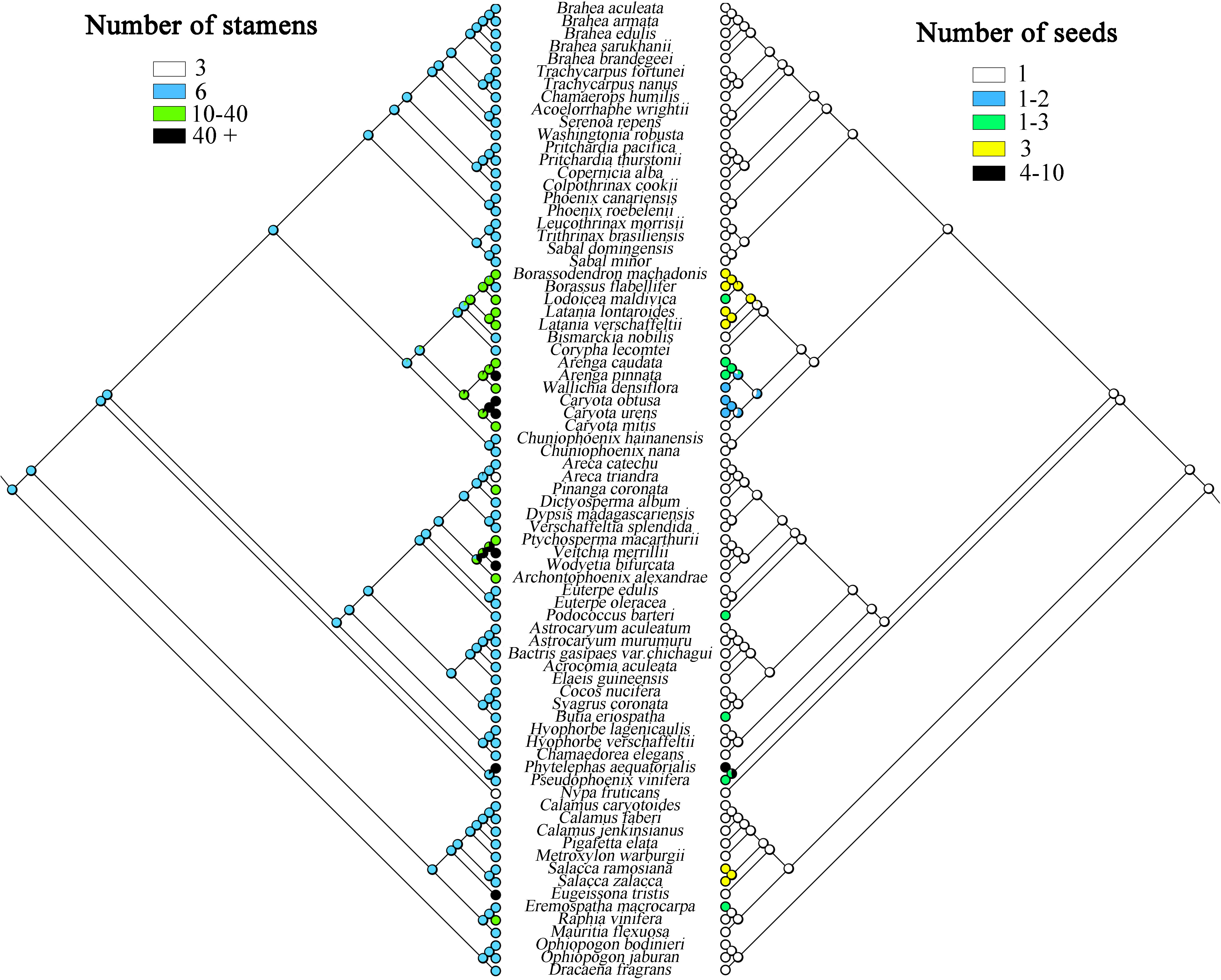
Figure 8 Maximum likelihood analysis of morphological traits evolution in Arecaceae based on the chloroplast data set. Left, Number of stamens; Right, Number of seeds.
Discussion
Plastome genome structure comparisons and sequence difference analysis
According to the comparative results of chloroplast genome structure (Figure 2; Table 2), the size of the 74 chloroplast genomes of Arecaceae range from 153,806-160,122 bp, of which LSC length was 82,900-87,639 bp, the SSC was 13,768-18,380 bp, and the IR was 26,007-28,228 bp. There were 76-82 unique protein-coding genes, 29-32 tRNA genes and four rRNA genes. The structural features of Arecaceae plastomes were similar to those of most other angiosperms (Palmer, 1991; Raubeson and Jansen, 2005; Chumley et al., 2006; Huang et al., 2016). Studies had shown that the chloroplast genome of land plants is a circular double-stranded DNA molecule ranging in size from 107-218 kb, which can vary from species to species (Daniell et al., 2016). In angiosperms, the chloroplast genome is usually between 120-160 kb in size, with sequences of the LSC between 80-90 kb, the SSC between 16-27 kb and the IR between 20-28 kb (Palmer and Delwiche, 1998; Raubeson and Jansen, 2005; Chumley et al., 2006; Zhang et al., 2012; Serrano et al., 2013). This indicates that the Arecaceae chloroplast genome size was within the normal range of angiosperm chloroplast genome size. According to our results, both the largest chloroplast genome (160,122 bp) and the smallest chloroplast genome (153,806 bp) were in Coryphoideae. The differences in chloroplast genome size among different subfamilies were primarily due to differences in the length of the IR region. Due to the contraction and expansion of IR region, there were differences between IR/SC boundaries of plastid genome (Kim and Lee, 2004; Chang et al., 2006; Raubeson et al., 2007). These changes may lead to gene loss or gain (Wicke et al., 2011; Wang et al., 2018), which are usually the main cause of changes in the size of the plastome (Kim and Lee, 2004; Wang and Messing, 2011; Zhang et al., 2016). In our results (Figure 4), the IR regions of Dictyosperma album in Arecoideae and Pseudophoenix vinifera in Ceroxyloideae were both contracted at the IRb/LSC boundary; the rps19 gene of Dictyosperma album was complete in the LSC region, while the length of rps19 in Pseudophoenix vinifera was 231 bp in the LSC region and 48 bp in the IRb region. The IR region of Eugeissona tristis in Calamoideae was expanded at the IRa/SSC boundary, and ycf1 expanded to 2366 bp in the IRa region, resulting in the loss of ndhF, making the IRb/SSC boundary rpl32. The phenomenon of expansion or contraction of the IR region (Chumley et al., 2006; Wang et al., 2008; Guisinger et al., 2010; Yang et al., 2010; Sun et al., 2013) and gene loss (Wicke et al., 2011; Barrett et al., 2014) had also been found in previous studies.
To analyze the different levels of gene sequences, this study used mVISTA to draw sequence identity plots (Figure 3). The results showed that the non-coding regions showed a higher level of divergence than the coding regions. Most of the sequence divergence was concentrated in the non-coding regions which were less conserved than the coding regions, similar to most chloroplast genomes of angiosperms (Perry and Wolfe, 2002; Huang et al., 2014; Zhang et al., 2016). In addition, the diversity variation in the IR region was smaller than that in the SC regions (LSC and SSC). The small variation in the IR region was primarily due to the duplication correction caused by gene conversion between IR sequences (Khakhlova and Bock, 2006). In conclusion, coding region and IR region showed higher conservation than non-coding region and SC region.
Phylogenetic relationships of Arecaceae
Compared to earlier phylogenetic studies of Arecaceae (Hahn, 2002a; Asmussen et al., 2006; Eiserhardt et al., 2011; Faurby et al., 2016), this study was the first to use plastome sequences for segmentation and data partitioning to analyze the phylogenetic relationship of Arecaceae. Based on the complete chloroplast genome, phylogenetic analysis of the seven datasets was performed using ML and BI analysis. The topological structure based on ML and BI analysis were highly consistent in each data set. Except for the SSC and IRb datasets, the phylogenetic relationships inferred from the other five datasets (whole chloroplast genome, LSC, No-IRa, CDS and Non-CDS) were consistent by strongly supporting the five subfamilies were monophyletic and the relationships within each subfamily (BS/PP = 100/1). Our results show similar relationships as in most other studies of Arecaceae (Asmussen et al., 2006, which relied on plastid DNA; Baker et al., 2009, which relied on plastid, nuclear ribosomal, and low-copy nuclear DNA; Faurby et al., 2016, which relied on morphological and genetic data) and strongly support a sister relationship between Ceroxyloideae and Arecoideae (BS/PP = 100/1). Furthermore, the relationships among the tribes/genera were well-supported based on the phylogenetic relationships of the whole chloroplast genome. However, the phylogenetic positions of tribes differ in different studies. In Arecoideae, the intertribal relationships in this study were consistent with those in Baker et al. (2009) and Faurby et al. (2016) (Chumaedoreae + [Coceae + [Oranieae + [Areceae + Euterpeae]]]), whereas Comer et al. (2015; 2016) inferred different relationships (Chumaedoreeae+[Oranieae+[Coceae+[Areceae+Euterpeae]]]). In Coryphoideae, Sabaleae+Cryosophileae and Trachycarpeae+Phoeniceae were sisters, while in Faurby et al. (2016) Sabaleae and [Cryosophileae+[Trachycarpeae+Phoeniceae]] were sisters, and in Baker et al. (2009) Phoeniceae was the sister group of [Trachycarpeae+[Sabalaee+Cryosophileae]]. The different studies cited above all used different data and methods, while the sampling was also not the same, so unsurprisingly different topologies were generated. In addition, the all-evidence species-level supertree of Faurby et al. (2016) and the complete genus-level supermatrix tree of Baker et al. (2009) showed great differences in the phylogenetic position of the intergeneric relationships within tribes, with the differences largely due to differences in taxa sampling. Here, this study lacks more species in some of the genera/tribes and cannot fully compare the relationship between all genera/tribes with previous studies. Notably, due to the small number of taxa sampled in this study, the relationships between tribes, especially intergeneric, are still not clear, which is a limitation. Therefore, the evolutionary relationships and division among the various tribes/genera still need further studying with expanded sampling and more molecular data.
Molecular dating
Correct phylogenetic relationships and estimates of divergence times are important for evolutionary studies. We selected the complete chloroplast genome dataset to facilitate and optimize the estimation of divergence time. Molecular dating results suggest that the diversification of Arecaceae most likely began 96.60 [84.90-107.60] Ma in the late Cretaceous. We infer that the crown age of Arecaceae (96.60 Ma) was younger than that estimated by Janssen and Bremer (2004; 110 Ma), Onstein et al. (2018; ca 110 Ma), older than that estimated by Mennes et al. (2015; 84-90 Ma) and Givnish et al. (2018; ca 85 Ma), and similar to the results estimated by Li et al. (2019; 96.2 Ma), Matsunaga and Smith (2021; ca 93 Ma), Couvreur et al. (2011; 100 Ma), and Baker and Couvreur (2013; 100 Ma). The differences in divergence time estimates between different studies may be due to factors such as the setting of fossil calibration points, taxon sampling, choice of molecular data, and different operating methods. Compared with other related studies (Janssen and Bremer, 2004; Couvreur et al., 2011; Baker and Couvreur, 2013; Mennes et al., 2015), this study selected two new suitable fossil node calibrations, and a secondary calibration point as the limit of the total root crown group time. Our findings were similar to those of most other studies, with the Arecaceae lineage originating in the Cretaceous and early Paleogene (Janssen and Bremer, 2004; Harley, 2006; Couvreur et al., 2011; Baker and Couvreur, 2013; Givnish et al., 2018; Matsunaga and Smith, 2021). The divergence times of subfamilies except Calamoideae were similar to the results of Couvreur et al., 2011; Baker and Couvreur (2013) (Nypoideae, stem, 93.5 Ma; Coryphoideae, stem, 86.6 Ma; Ceroxyloideae, stem, 78.2 Ma; Arecoideae, crown, 73.6 Ma). The crown age of Calamoideae in these two studies was 80.2 Ma, while the study by Baker and Dransfield (2000) also supported the early fossil record of Calamus originating in Gondwana, and Hartwich et al. (2010) found the large palm fossil of late Eocene also suggesting that Calamoideae was distributed early in Gondwana. However, our results (53.39 Ma) were quite different from those studies and therefore need to be interpreted with caution. Different studies run different generations with BEAST, resulting in different crown-group results. Our results infer that Ceroxyloideae originated in Gondwana during the Cretaceous, which was similar to the findings of Khan et al. (2020) which showed that the Ceroxyloideae diverged at the Cretaceous-Paleogene boundary of central India (ca 66-65 Ma) and were present in India about 10-15 million years before the collision between India with Eurasia. After the collision, the group may have dispersed in East Asia, North America, and reached South America during the Miocene. Our dated phylogenetic relationships indicate that the major lineages of Arecaceae diverged during the Late Cretaceous and underwent rapid speciation events from the Paleocene to Eocene, with widespread distributions in the Eocene. The palm radiation primarily occurred in the Early Cenozoic, and with the warming of the climate and the passage of time, the species diversity gradually increased (Daghlian, 1981; Kvaček and Herman, 2004); while the species diversity and distribution range decreased with the cooling in the Oligocene and Late Miocene (Daghlian, 1981; Harley, 2006), suggesting that species diversity changes were related to climate, meanwhile indicating the existence of a subtropical to tropical paleoclimate in the Late Eocene (Hartwich et al., 2010).
Morphological evolution of species
In this study, we used several traits of Arecaceae, stamen number, seed number, plant type, and pericarp type to reconstruct ancestral traits. The results of reconstructed ancestral traits show that a smooth pericarp is the likely ancestral trait of the family. For this trait, we infer that the smooth fruit pericarp gradually evolved into spiny, imbricate scales, and rough pericarp. There is extensive homogeneity in morphological characters among species of Arecaceae. For example, in Calamoideae, the fruit pericarp is covered with scales, whereas in other subfamilies most of the fruit pericarps are smooth except for a few species. Although there is clear convergent evolution of fruit structure diversity and many traits in Arecaceae, fruit traits can still be strongly taxonomically distinct below the subfamily level (Moore and Uhl, 1982). Similarly, the fruits of Calamoideae are most easily distinguished from other subfamilies. Moore and Uhl (1982) also showed the evolution of fruit development in Arecaceae from fleshy to dry and fibrous fruits. For the trait of plant type, the results indicate that monoecy may be the ancestral trait of the family. In angiosperms, hermaphroditism is considered to be the ancestral state (Endress and Doyle, 2009). Weiblen et al. (2000) inferred that the ancestral trait of monocotyledons was hermaphroditic and concluded that dioecy from hermaphroditism underwent transformations more frequently than that from monoecy, and that reversals from dioecy to monoecy also occured. In addition, the transition model and mechanism from hermaphroditism to dioecy may be through a transient gynodioecious phase. As shown in De Jong et al. (2008) in a model of sex allocation at the flower level, a possible pathway for the transition from hermaphroditism to monoecy is through andromonoecy. Renner and Ricklefs (1995) indicated that dioecy may have evolved from monoecy through different adjustments in flower sex ratios among individual plants. Moore and Uhl (1982) showed that Arecaceae evolved from hermaphroditism to monoecism and later with polygamy or monoecism to dioecism. In Arecoideae, the largest subfamily of Arecaceae, species are overwhelmingly monoecious, while only Chamaedorea in the tribe Chamaedoreeae evolved as a dioecious plant. Castaño et al. (2014) considered that dioecy has evolved twice independently from a monoecious ancestor in this tribe, and the genus Chamaedorea exhibits high variability in reproductive morphology. In this case, the origin of Arecaceae plants was ambiguous, and Arecaceae was simply rated as a family that has both monoecy and dioecy.
The ancestral state reconstructions indicate that a seed number of one is the ancestral trait in Arecaceae. Seeds are relatively stable during evolution, with only a few evolving to vary from one to three seeds, and even four to 10 seeds in Phytelephas of Ceroxyloideae. This occurrence of many seeds may be related to fruit formation. In Phytelephas, the fruit is in clusters, consisting of multiple single fruits, which may contain multiple seeds; in the tribe Borasseae, the endocarp of the fruit is composed of three separate hard pyrenes, and the seeds may be numbered one to three (Dransfield et al., 2008). Independent evolutionary events may exist in Arecoideae, with species in the tribe Coceae evolving one to three seeds in varying numbers. The size of seeds may be influenced by the size and structure of the plant and is a major determinant of seed dispersal, seedling growth, and plant evolution (Moles, 2018).
The number of stamens in Arecaceae shows a wide diversity, with numbers ranging from dozens to hundreds (Table 5). In the study, the number of stamens in ancestral species of the Arecaceae may have been six, while later evolving to more numerous stamens. The number of stamens in Coryphoideae and Arecoideae species are more diverse relative to other subfamily species. Phytelephas, in Ceroxyloideae, possesses both numerous stamens and dioecious species. The number of stamens in this genus varies from 36 to more than 900 stamens, which is the highest number known in the family (Uhl and Moore, 1977; Dransfield et al., 2008). The number of stamens may be related to the expansion and morphological changes in the apical part of the flower prior to germination, and stamen centrifugal development appears to be a method of apical expansion to accommodate the increased number of stamens (Uhl and Moore, 1977). In Calamoideae, species of Eugeissona have a large number of stamens, which can upwards of 70. Stauffer et al. (2016) showed that Eugeissona exhibits a range of reproductive characteristics that are generally unique among the early differentiating subfamilies, and that the pistil of this genus have an unusual structure in terms of carpel fusion and differentiation for the Aeacaceae. Nypa in Nypoideae and Areca in Arecoideae showed degeneration in the number of stamens, which was reduced to three. The number of stamens in Nypa was influenced by its morphology and the vascular system, with filaments innately fused and anthers fused adaxially to the connectives (Uhl, 1972). In any case, the decrease or increase in the number of stamens represents a state of derivation.
Conclusions
In this study, we assembled the complete chloroplast genomes of 24 Arecaceae species, providing a genomic resource for future research. To better understand Arecaceae, we analyzed and compared the chloroplast genome structural features of Arecaceae, inferred phylogenetic relationships, estimated the divergence time of Arecaceae, and reconstructed the analysis of ancestral traits. Based on the phylogenetic relationships of the whole plastome and multiple datasets analyzed by ML/BI, all five subfamilies were supported as monophyletic, the relationship between subfamilies was strongly supported, and the relationship between some tribes/genera was also well support. In addition, the estimation of the divergence time of Arecaceae shows that the crown age of Arecaceae was 96.60 [84.90-107.60] Ma in the Late Cretaceous, and the stem age was 102.40 [93.44–111.17] Ma. Through the analysis of the ancestral traits of Arecaceae, we can infer that the ancestral form was monoecious, with a single seed, six stamens, and a smooth pericarp. The chloroplast genome resources obtained in this study will be helpful for future studies on species identification and evolution, genetic diversity, and phylogeny of Arecaceae. However, the phylogenetic analyses of this study still had had some limitations. Future studies need to expand the acquisition of samples, and increase the data availability of whole chloroplast genomes, and use nuclear data to support the inferred relationships on a large scale. To this end, we can more comprehensively analyze and discuss the phylogeny and evolution of Arecaceae.
Data availability statement
The datasets presented in this study can be found in online repositories. The names of the repository/repositories and accession number(s) can be found in the article/Supplementary Material.
Author contributions
D-JC performed all molecular experiments, analyzed the data, and wrote the manuscript; H-XW, Q-HS, QW assisted in analyzing the data; JL helped run the data analysis and revise the manuscript; H-FW conceived and directed the study and revised the manuscript. All authors contributed to the article and approved the submitted version.
Funding
This study was funded by Hainan Province Science and Technology Special Fund (ZDYF2022XDNY190), the Project of Sanya Yazhou Bay Science and Technology City (Grant number: SCKJ-JYRC-2022-83, HNF202222), and Hainan Provincial Natural Science Foundation of China (421RC486).
Acknowledgments
We are sincerely thank H-XW, Q-HS, L-YG, X-FZ, X-RK, J-HW and X-LC for their kind help and experimental samples in this study, as well as for their help in molecular experiments and data analysis; thanks to Drs. L-XG and QC for their help in species identification; and thanks to H-FW and JL for their unique comments and suggestions, and for improving the manuscript; meanwhile thanks to the reviewers for their thoughtful comments and suggestions to us.
Conflict of interest
Author QW is employed by Hainan Shengda Modern Agriculture Development Co., Ltd., Qionghai, China.
The remaining authors declare that the research was conducted in the absence of any commercial or financial relationships that could be construed as a potential conflict of interest.
Publisher’s note
All claims expressed in this article are solely those of the authors and do not necessarily represent those of their affiliated organizations, or those of the publisher, the editors and the reviewers. Any product that may be evaluated in this article, or claim that may be made by its manufacturer, is not guaranteed or endorsed by the publisher.
Supplementary material
The Supplementary Material for this article can be found online at: https://www.frontiersin.org/articles/10.3389/fpls.2022.960588/full#supplementary-material
Supplementary Figure S1 | Multiple genome alignments visualization of chloroplast genome sequence in Arecaceae.
Supplementary Figures S2–7 | The S2–S7 are the phylogenetic relationships obtained by ML and BI analysis. Based on six datasets such as LSC, SSC, IRB, NO-IRa, CDS, and Non-CDS. “*” means 100%/1.0 support value, “-” means bootstrap value/Bayesian posterior probability less than 60/0.6. Different colors represent different clades of subfamilies.
Abbreviations
BI, Bayesian Inference; CTAB, Cetyltrimethylammonium bromide; IR, Inverted repeat; LSC, Large single copy; GTR, General time reversible; ML, Maximum Likelihood; PI, Phylogenetic informativeness; rRNA, Ribosomal RNA; SSC, Small single copy; tRNA, Transfer RNA.
References
Angiosperm Phylogeny Group, Chase, M. W., Christenhusz, M. J., Fay, M. F., Byng, J. W., Judd, W. S., et al. (2016). An update of the angiosperm phylogeny group classification for the orders and families of flowering plants: APG IV. Bot. J. Linn. Soc. 181 (1), 1–20. doi: 10.1111/boj.12385
Asmussen, C. B., Baker, W. J., Dransfield, J. (2000). Phylogeny of the palm family (Arecaceae) based on rps16 intron and trnL-trnF plastid DNA sequences. Monocots.: Syst. Evol., 525–535. Available at: https://www.researchgate.net/publication/215898335
Asmussen, C. B., Dransfield, J., Deickmann, V., Barfod, A. S., Pintaud, J. C., Baker, W. J. (2006). A new subfamily classification of the palm family (Arecaceae): evidence from plastid DNA phylogeny. Bot. J. Linn. Soc. 151 (1), 15–38. doi: 10.1111/j.1095-8339.2006.00521.x
Bacon, C. D., Velásquez-Puentes, F., Flórez-Rodríguez, A., Balslev, H., Galeano, G., Bernal, R., et al. (2016). Phylogenetics of iriarteeae (Arecaceae), cross-Andean disjunctions and convergence of clustered infructescence morphology in wettinia. Bot. J. Linn. Soc. 182 (2), 272–286. doi: 10.1111/boj.12421
Baker, W. J., Couvreur, T. L. (2013). Global biogeography and diversification of palms sheds light on the evolution of tropical lineages. i. historical biogeography. J. Biogeogr. 40 (2), 274–285. doi: 10.1111/j.1365-2699.2012.02795.x
Baker, W. J., Dransfield, J. (2000). Towards a biogeographic explanation of the calamoid palms. Monocots: systematics and evolution 545–553. https://www.researchgate.net/publication/215898342
Baker, W. J., Dransfield, J. (2016). Beyond genera palmarum: progress and prospects in palm systematics. Bot. J. Linn. Soc. 182 (2), 207–233. doi: 10.1111/boj.12401
Baker, W. J., Norup, M. V., Clarkson, J. J., Couvreur, T. L., Dowe, J. L., Lewis, C. E., et al. (2011). Phylogenetic relationships among arecoid palms (Arecaceae: Arecoideae). Ann. Bot. 108 (8), 1417–1432. doi: 10.1093/aob/mcr020
Baker, W. J., Savolainen, V., Asmussen-Lange, C. B., Chase, M. W., Dransfield, J., Forest, F., et al. (2009). Complete generic-level phylogenetic analyses of palms (Arecaceae) with comparisons of supertree and supermatrix approaches. Syst. Biol. 58 (2), 240–256. doi: 10.1093/sysbio/syp021
Barrett, C. F., Freudenstein, J. V., Li, J., Mayfield-Jones, D. R., Perez, L., Pires, J. C., et al. (2014). Investigating the path of plastid genome degradation in an early-transitional clade of heterotrophic orchids, and implications for heterotrophic angiosperms. Mol. Biol. Evol. 31 (12), 3095–3112. doi: 10.1093/molbev/msu252
Barrett, C. F., Sinn, B. T., King, L. T., Medina, J. C., Bacon, C. D., Lahmeyer, S. C., et al. (2019). Phylogenomics, biogeography and evolution in the American genus brahea (Arecaceae). Bot. J. Linn. Soc. 190 (3), 242–259. doi: 10.1093/botlinnean/boz015
Castaño, F., Stauffer, F., Marquinez, X., Crèvecoeur, M., Collin, M., Pintaud, J. C., et al. (2014). Floral structure and development in the monoecious palm Gaussia attenuata (Arecaceae; arecoideae). Ann. Bot. 114 (7), 1483–1495. doi: 10.1093/aob/mcu133
Chang, C. C., Lin, H. C., Lin, I. P., Chow, T. Y., Chen, H. H., Chen, W. H., et al. (2006). The chloroplast genome of Phalaenopsis aphrodite (Orchidaceae): comparative analysis of evolutionary rate with that of grasses and its phylogenetic implications. Mol. Biol. Evol. 23 (2), 279–291. doi: 10.1093/molbev/msj029
Chumley, T. W., Palmer, J. D., Mower, J. P., Fourcade, H. M., Calie, P. J., Boore, J. L., et al. (2006). The complete chloroplast genome sequence of Pelargonium× hortorum: organization and evolution of the largest and most highly rearranged chloroplast genome of land plants. Mol. Biol. Evol. 23 (11), 2175–2190. doi: 10.1093/molbev/msl089
Comer, J. R., Zomlefer, W. B., Barrett, C. F., Davis, J. I., Stevenson, D. W., Heyduk, K., et al. (2015). Resolving relationships within the palm subfamily arecoideae (Arecaceae) using plastid sequences derived from next-generation sequencing. Am. J. Bot. 102 (6), 888–899. doi: 10.3732/ajb.1500057
Comer, J. R., Zomlefer, W. B., Barrett, C. F., Stevenson, D. W., Heyduk, K., Leebens-Mack, J. H. (2016). Nuclear phylogenomics of the palm subfamily arecoideae (Arecaceae). Mol. Phylogenet. Evol. 97, 32–42. doi: 10.1016/j.ympev.2015.12.015
Couvreur, T. L., Forest, F., Baker, W. J. (2011). Origin and global diversification patterns of tropical rain forests: inferences from a complete genus-level phylogeny of palms. BMC Biol. 9 (1), 1–12. doi: 10.1186/1741-7007-9-44
Daghlian, C. P. (1981). A review of the fossil record of monocotyledons. Bot. Rev. 47 (4), 517–555. doi: 10.1007/BF02860540
Daniell, H., Lin, C. S., Yu, M., Chang, W. J. (2016). Chloroplast genomes: diversity, evolution, and applications in genetic engineering. Genome Biol. 17 (1), 1–29. doi: 10.1186/s13059-016-1004-2
Darling, A. C., Mau, B., Blattner, F. R., Perna, N. T. (2004). Mauve: multiple alignment of conserved genomic sequence with rearrangements. Genome Res. 14 (7), 1394–1403. doi: 10.1101/gr.2289704
De Jong, T. J., Shmida, A., Thuijsman, F. (2008). Sex allocation in plants and the evolution of monoecy. Evol. Ecol. Res. 10 (8), 1087–1109.
de Souza Magnabosco, J. W., de Freitas Fraga, H. P., da Silva, R. S., Rogalski, M., de Souza, E. M., Guerra, M. P., et al. (2020). Characterization of the complete plastid genome of butia eriospatha (Arecaceae). Genet. Mol. Biol. 43 (4). doi: 10.1590/1678-4685-GMB-2020-0023
Doyle, J. J., Doyle, J. L. (1987). A rapid DNA isolation procedure for small quantities of fresh leaf tissue. Phytochem. Bull. 19, 11–15.
Dransfield, J., Uhl, N. W., Asmussen, C. B., Baker, W. J., Harley, M. M., Lewis, C. E. (2005). A new phylogenetic classification of the palm family, Arecaceae. Kew Bull., 559–569. Available at: https://www.jstor.org/stable/25070242
Dransfield, J., Uhl, N. W., Asmussen, C. B., Baker, W. J., Harley, M. M., Lewis, C. E. (2008). Genera palmarum-the evolution and classification of the palms. Royal Botanic Gardens, Kew, 732 pp. doi: 10.34885/92
Drummond, A. J., Suchard, M. A., Xie, D., Rambaut, A. (2012). Bayesian Phylogenetics with BEAUti and the BEAST 1.7. Mol. Biol. Evol. 29 (8), 1969–1973. doi: 10.1093/molbev/mss075
Eiserhardt, W. L., Pintaud, J. C., Asmussen-Lange, C., Hahn, W. J., Bernal, R., Balslev, H., et al. (2011). Phylogeny and divergence times of bactridinae (Arecaceae, palmae) based on plastid and nuclear DNA sequences. Taxon 60 (2), 485–498. doi: 10.1002/tax.602016
Endress, P. K., Doyle, J. A. (2009). Reconstructing the ancestral angiosperm flower and its initial specializations. Am. J. Bot. 96 (1), 22–66. doi: 10.3732/ajb.0800047
Fadini, R. F., Fleury, M., Donatti, C. I., Galetti, M. (2009). Effects of frugivore impoverishment and seed predators on the recruitment of a keystone palm. Acta Oecol. 35 (2), 188–196. doi: 10.1016/j.actao.2008.10.001
Faurby, S., Eiserhardt, W. L., Baker, W. J., Svenning, J. C. (2016). An all-evidence species-level supertree for the palms (Arecaceae). Mol. Phylogenet. Evol. 100, 57–69. doi: 10.1016/j.ympev.2016.03.002
Faye, A., Pintaud, J. C., Baker, W. J., Sonké, B., Couvreur, T. L. P. (2014). A plastid phylogeny of the African rattans (Ancistrophyllinae, arecaceae). Syst. Bot. 39 (4), 1099–1107. doi: 10.1600/036364414X682634
Frazer, K. A., Pachter, L., Poliakov, A., Rubin, E. M., Dubchak, I. (2004). VISTA: computational tools for comparative genomics. Nucleic Acids Res. 32 (suppl_2), W273–W279. doi: 10.1093/nar/gkh458
Givnish, T. J., Zuluaga, A., Spalink, D., Soto Gomez, M., Lam, V. K., Saarela, J. M., et al. (2018). Monocot plastid phylogenomics, timeline, net rates of species diversification, the power of multi-gene analyses, and a functional model for the origin of monocots. Am. J. Bot. 105 (11), 1888–1910. doi: 10.1002/ajb2.1178
Guisinger, M. M., Chumley, T. W., Kuehl, J. V., Boore, J. L., Jansen, R. K. (2010). Implications of the plastid genome sequence of typha (Typhaceae, poales) for understanding genome evolution in poaceae. J. Mol. Evol. 70 (2), 149–166. doi: 10.1007/s00239-009-9317-3
Hahn, W. J. (2002a). A molecular phylogenetic study of the palmae (Arecaceae) based on atpB, rbcL, and 18S nrDNA sequences. Syst. Biol. 51 (1), 92–112. doi: 10.1080/106351502753475899
Hahn, W. J. (2002b). A phylogenetic analysis of the arecoid line of palms based on plastid DNA sequence data. Mol. Phylogenet. Evol. 23 (2), 189–204. doi: 10.1016/S1055-7903(02)00022-2
Harley, M. M. (2006). A summary of fossil records for arecaceae. Bot. J. Linn. Soc. 151 (1), 39–67. doi: 10.1111/j.1095-8339.2006.00522.x
Hartwich, S. J., Conran, J. G., Bannister, J. M., Lindqvist, J. K., Lee, D. E. (2010). Calamoid fossil palm leaves and fruits (Arecaceae: Calamoideae) from late Eocene southland, new Zealand. Aust. Syst. Bot. 23 (2), 131–140. doi: 10.1071/SB09027
Heyduk, K., Trapnell, D. W., Barrett, C. F., Leebens-Mack, J. (2016). Phylogenomic analyses of species relationships in the genus sabal (Arecaceae) using targeted sequence capture. Biol. J. Linn. Soc. 117 (1), 106–120. doi: 10.1111/bij.12551
Hoang, D. T., Chernomor, O., Von Haeseler, A., Minh, B. Q., Vinh, L. S. (2018). UFBoot2: improving the ultrafast bootstrap approximation. Mol. Biol. Evol. 35 (2), 518–522. doi: 10.1093/molbev/msx281
Horn, J. W., Fisher, J. B., Tomlinson, P. B., Lewis, C. E., Laubengayer, K. (2009). Evolution of lamina anatomy in the palm family (Arecaceae). Am. J. Bot. 96 (8), 1462–1486. doi: 10.3732/ajb.0800396
Huang, H., Shi, C., Liu, Y., Mao, S. Y., Gao, L. Z. (2014). Thirteen Camelliachloroplast genome sequences determined by high-throughput sequencing: genome structure and phylogenetic relationships. BMC Evol. Biol. 14 (1), 1–17. doi: 10.1186/1471-2148-14-151
Huang, J., Zhang, C., Zhao, X., Fei, Z., Wan, K., Zhang, Z., et al. (2016). The jujube genome provides insights into genome evolution and the domestication of sweetness/acidity taste in fruit trees. PloS Genet. 12 (12), e1006433. doi: 10.1371/journal.pgen.1006433
Janssen, T., Bremer, K. (2004). The age of major monocot groups inferred from 800+ rbcL sequences. Bot. J. Linn. Soc. 146 (4), 385–398. doi: 10.1111/j.1095-8339.2004.00345.x
Jin, J. J., Yu, W. B., Yang, J. B., Song, Y., DePamphilis, C. W., Yi, T. S., et al. (2020). GetOrganelle: a fast and versatile toolkit for accurate de novo assembly of organelle genomes. Genome Biol. 21 (1), 1–31. doi: 10.1186/s13059-020-02154-5
Johnson, D. V. (2010). Tropical palms—2010 revision. Food and Agriculture Organization of the United Nations, Rome.
Joseph, D., George, J., Mathews, M. M., Mathew, F., Varghese, B., Sunny, B. (2019). A compilation on anti-diabetic profile of Cocos nucifera. Res. J. Pharm. Technol. 12 (8), 3791–3796. doi: 10.5958/0974-360X.2019.00649.8
Katoh, K., Standley, D. M. (2013). MAFFT multiple sequence alignment software version 7: improvements in performance and usability. Mol. Biol. Evol. 30 (4), 772–780. doi: 10.1093/molbev/mst010
Kearse, M., Moir, R., Wilson, A., Stones-Havas, S., Cheung, M., Sturrock, S., et al. (2012). Geneious basic: an integrated and extendable desktop software platform for the organization and analysis of sequence data. Bioinformatics 28 (12), 1647–1649. doi: 10.1093/bioinformatics/bts199
Khakhlova, O., Bock, R. (2006). Elimination of deleterious mutations in plastid genomes by gene conversion. Plant J. 46 (1), 85–94. doi: 10.1111/j.1365-313X.2006.02673.x
Khan, M. A., Hazra, M., Mahato, S., Spicer, R. A., Roy, K., Hazra, T., et al. (2020). A Cretaceous gondwana origin of the wax palm subfamily (Ceroxyloideae: Arecaceae) and its paleobiogeographic context. Rev. Palaeobot. Palynol. 283, 104318. doi: 10.1016/j.revpalbo.2020.104318
Kim, K. J., Lee, H. L. (2004). Complete chloroplast genome sequences from Korean ginseng (Panax schinseng nees) and comparative analysis of sequence evolution among 17 vascular plants. DNA Res. 11 (4), 247–261. doi: 10.1093/dnares/11.4.247
Kissling, W. D., Balslev, H., Baker, W. J., Dransfield, J., Göldel, B., Lim, J. Y., et al. (2019). PalmTraits 1.0, a species-level functional trait database of palms worldwide. Sci. Data 6 (1), 1–13. doi: 10.1038/s41597-019-0189-0
Kvaček, J., Herman, A. B. (2004). Monocotyledons from the early campanian (Cretaceous) of grünbach, lower Austria. Rev. Palaeobot. Palynol. 128 (3-4), 323–353. doi: 10.1016/S0034-6667(03)00154-4
Lanfear, R., Calcott, B., Ho, S. Y., Guindon, S. (2012). PartitionFinder: combined selection of partitioning schemes and substitution models for phylogenetic analyses. Mol. Biol. Evol. 29 (6), 1695–1701. doi: 10.1093/molbev/mss020
Li, H. T., Yi, T. S., Gao, L. M., Ma, P. F., Zhang, T., Yang, J. B., et al. (2019). Origin of angiosperms and the puzzle of the Jurassic gap. Nat. Plants 5 (5), 461–470. doi: 10.1038/s41477-019-0421-0
Lohse, M., Drechsel, O., Kahlau, S., Bock, R. (2013). OrganellarGenomeDRAW–a suite of tools for generating physical maps of plastid and mitochondrial genomes and visualizing expression data sets. Nucleic Acids Res. 41 (W1), W575–W581. doi: 10.1093/nar/gkt289
Maddison, W. P. (2008). Mesquite: A modular system for evolutionary analysis. Evol. 62, 1103–1118. Available at: http://mesquiteproject.org
Matsunaga, K. K., Smith, S. Y. (2021). Fossil palm reading: using fruits to reveal the deep roots of palm diversity. Am. J. Bot. 108 (3), 472–494. doi: 10.1002/ajb2.1616
Mennes, C. B., Lam, V. K., Rudall, P. J., Lyon, S. P., Graham, S. W., Smets, E. F., et al. (2015). Ancient gondwana break-up explains the distribution of the mycoheterotrophic family corsiaceae (Liliales). J. Biogeogr. 42 (6), 1123–1136. doi: 10.1111/jbi.12486
Moles, A. T. (2018). Being John harper: Using evolutionary ideas to improve understanding of global patterns in plant traits. J. Ecol. 106 (1), 1–18. doi: 10.1111/1365-2745.12887
Moore, H. E., Uhl, N. W. (1982). Major trends of evolution in palms. Bot. Rev. 48 (1), 1–69. doi: 10.1007/bf02860535
Nguyen, L. T., Schmidt, H. A., Von Haeseler, A., Minh, B. Q. (2015). IQ-TREE: a fast and effective stochastic algorithm for estimating maximum-likelihood phylogenies. Mol. Biol. Evol. 32 (1), 268–274. doi: 10.1093/molbev/msu300
Onstein, R. E., Baker, W. J., Couvreur, T. L., Faurby, S., Herrera-Alsina, L., Svenning, J. C., et al. (2018). To adapt or go extinct? the fate of megafaunal palm fruits under past global change. Proc. R. Soc. B. 285 (1880), 20180882. doi: 10.1098/rspb.2018.0882
Palmer, J. D. (1991). Plastid chromosomes: structure and evolution. Mol. Biol. Plastids. 7, 5–53. doi: 10.1016/B978-0-12-715007-9.50009-8
Palmer, J. D., Delwiche, C. F. (1998). “The origin and evolution of plastids and their genomes,” in Molecular systematics of plants II (Boston, MA: Springer), 375–409. doi: 10.1007/978-1-4615-5419-6_13
Perry, A. S., Wolfe, K. H. (2002). Nucleotide substitution rates in legume chloroplast DNA depend on the presence of the inverted repeat. J. Mol. Evol. 55 (5), 501–508. doi: 10.1007/PL00020998
Pichardo-Marcano, F. J., Nieto-Blázquez, M. E., MacDonald, A. N., Galeano, G., Roncal, J. (2019). Phylogeny, historical biogeography and diversification rates in an economically important group of Neotropical palms: Tribe euterpeae. Mol. Phylogenet. Evol. 133, 67–81. doi: 10.1016/j.ympev.2018.12.030
Posada, D. (2008). jModelTest: phylogenetic model averaging. Mol. Biol. Evol. 25 (7), 1253–1256. doi: 10.1093/molbev/msn083
Rambaut, A., Drummond, A. J., Xie, D., Baele, G., Suchard, M. A. (2018). Posterior summarization in Bayesian phylogenetics using tracer 1.7. Syst. Biol. 67 (5), 901–904. doi: 10.1093/sysbio/syy032
Raubeson, L. A., Jansen, R. K. (2005). Chloroplast genomes of plants. CABI Books. CABI International. doi: 10.1079/9780851999043.0045
Raubeson, L. A., Peery, R., Chumley, T. W., Dziubek, C., Fourcade, H. M., Boore, J. L., et al. (2007). Comparative chloroplast genomics: analyses including new sequences from the angiosperms nuphar advena and ranunculus macranthus. BMC Genomics 8 (1), 1–27. doi: 10.1186/1471-2164-8-174
Renner, S. S., Ricklefs, R. E. (1995). Dioecy and its correlates in the flowering plants. Am. J. Bot. 82 (5), 596–606. doi: 10.1002/j.1537-2197.1995.tb11504.x
Ronquist, F., Teslenko, M., van der Mark, P., Ayres, D. L., Darling, A., Höhna, S., et al. (2012). MrBayes 3.2: efficient Bayesian phylogenetic inference and model choice across a large model space. Syst. Biol. 61 (3), 539–542. doi: 10.1093/sysbio/sys029
Rose, R., Golosova, O., Sukhomlinov, D., Tiunov, A., Prosperi, M. (2019). Flexible design of multiple metagenomics classification pipelines with UGENE. Bioinformatics 35 (11), 1963–1965. doi: 10.1093/bioinformatics/bty901
Santorum, J. M., Darriba, D., Taboada, G. L., Posada, D. (2014). Jmodeltest. org: selection of nucleotide substitution models on the cloud. Bioinformatics 30 (9), 1310–1311. doi: 10.1093/bioinformatics/btu032
Schattner, P., Brooks, A. N., Lowe, T. M. (2005). The tRNAscan-SE, snoscan and snoGPS web servers for the detection of tRNAs and snoRNAs. Nucleic Acids Res. 33 (suppl_2), W686–W689. doi: 10.1093/nar/gki366
Serrano, M., Wang, B., Aryal, B., Garcion, C., Abou-Mansour, E., Heck, S., et al. (2013). Export of salicylic acid from the chloroplast requires the multidrug and toxin extrusion-like transporter EDS5. Plant Physiol. 162 (4), 1815–1821. doi: 10.1104/pp.113.218156
Smith, S. A., Dunn, C. W. (2008). Phyutility: a phyloinformatics tool for trees, alignments and molecular data. Bioinformatics 24 (5), 715–716. doi: 10.1093/bioinformatics/btm619
Stauffer, F. W., Siegert, S., Silberbauer-Gottsberger, I., Gottsberger, G. (2016). Floral structure in the Asian palm Eugeissona tristis Griff.(Arecaceae: Calamoideae), and description of a new nectary type in the family. Plant Syst. Evol. 302 (6), 629–639. doi: 10.1007/s00606-016-1292-8
Sun, Y. X., Moore, M. J., Meng, A. P., Soltis, P. S., Soltis, D. E., Li, J. Q., et al. (2013). Complete plastid genome sequencing of trochodendraceae reveals a significant expansion of the inverted repeat and suggests a paleogene divergence between the two extant species. PloS One 8 (4), e60429. doi: 10.1371/journal.pone.0060429
Tang, D., Liu, M., Ma, C., Wang, B., Wei, K. (2018). The greeing application of palm plants in jinghong city. J. Southwest. Forestry. Univ. (Social. Sciences). 2, 78–84. doi: CNKI:SUN:YNLS.0.2018-02-018
Thomas, R., De Franceschi, D. (2013). Palm stem anatomy and computer-aided identification: the coryphoideae (Arecaceae). Am. J. Bot. 100 (2), 289–313. doi: 10.3732/ajb.1200242
Trias-Blasi, A., Baker, W. J., Haigh, A. L., Simpson, D. A., Weber, O., Wilkin, P. (2015). A genus-level phylogenetic linear sequence of monocots. Taxon 64 (3), 552–581. doi: 10.12705/643.9
Uhl, N. W. (1972). Inflorescence and flower structure in Nypa fruticans (Palmae). Am. J. Bot. 59 (7), 729–743. doi: 10.1002/j.1537-2197.1972.tb10146.x
Uhl, N. W., Moore, J. H.E. (1977). Centrifugal stamen initiation in phytelephantoid palms. Am. J. Bot. 64 (9), 1152–1161. doi: 10.1002/j.1537-2197.1977.tb10805.x
Wang, C. L. (2007). Molecular phylogenetics of Chinese palmae based on nrDNA lTS and cpDNA matK sequence data. (Xiamen University). (07), 77. doi: CNKI:CDMD:2.2008.056847
Wang, R. J., Cheng, C. L., Chang, C. C., Wu, C. L., Su, T. M., Chaw, S. M. (2008). Dynamics and evolution of the inverted repeat-large single copy junctions in the chloroplast genomes of monocots. BMC Evol. Biol. 8 (1), 1–14. doi: 10.1186/1471-2148-8-36
Wang, W., Chen, S., Zhang, X. (2018). Whole-genome comparison reveals divergent IR borders and mutation hotspots in chloroplast genomes of herbaceous bamboos (Bambusoideae: Olyreae). Molecules 23 (7), 1537. doi: 10.3390/molecules23071537
Wang, W., Messing, J. (2011). High-throughput sequencing of three lemnoideae (duckweeds) chloroplast genomes from total DNA. PloS One 6 (9), e24670. doi: 10.1371/journal.pone.0024670
Wang, T., Ye, M. Y., Zhou, C. (2014). Application and analysis of arecaceae in tropical garden landscape of haikou city park. Modern. Horticult. 11), 13–17. doi: 10.14051/j.cnki.xdyy.2014.11.006
Weiblen, G. D., Oyama, R. K., Donoghue, M. J. (2000). Phylogenetic analysis of dioecy in monocotyledons. Am. Nat. 155 (1), 46–58. doi: 10.1086/303303
Wicke, S., Schneeweiss, G. M., Depamphilis, C. W., Müller, K. F., Quandt, D. (2011). The evolution of the plastid chromosome in land plants: gene content, gene order, gene function. Plant Mol. Biol. 76 (3), 273–297. doi: 10.1007/s11103-011-9762-4
Wick, R. R., Schultz, M. B., Zobel, J., Holt, K. E. (2015). Bandage: interactive visualization of de novo genome assemblies. Bioinformatics 31 (20), 3350–3352. doi: 10.1093/bioinformatics/btv383
Yang, M., Zhang, X., Liu, G., Yin, Y., Chen, K., Yun, Q., et al. (2010). The complete chloroplast genome sequence of date palm (Phoenix dactylifera l.). PloS One 5 (9), e12762. doi: 10.1371/journal.pone.0012762
Yang, J. P., Zhu, Z. L., Fan, Y. J., Zhu, F., Chen, Y. J., Niu, Z. T., et al. (2020). Comparative plastomic analysis of three bulbophyllum medicinal plants and its significance in species identification. Acta Pharm. Sin., 2736–2745. doi: 10.16438/j.0513-4870.2020-0967
Zhang, Y., Du, L., Liu, A., Chen, J., Wu, L., Hu, W., et al. (2016). The complete chloroplast genome sequences of five Epimedium species: lights into phylogenetic and taxonomic analyses. Front. Plant Sci. 7. doi: 10.3389/fpls.2016.00306
Keywords: Arecaceae, chloroplast genome, phylogeny, adaptive evolution, molecular dating
Citation: Chen D-J, Landis JB, Wang H-X, Sun Q-H, Wang Q and Wang H-F (2022) Plastome structure, phylogenomic analyses and molecular dating of Arecaceae. Front. Plant Sci. 13:960588. doi: 10.3389/fpls.2022.960588
Received: 03 June 2022; Accepted: 08 September 2022;
Published: 27 September 2022.
Edited by:
Michelle Waycott, University of Adelaide, AustraliaReviewed by:
Terezie Mandakova, Masaryk University, Czech RepublicYunheng Ji, Kunming Institute of Botany (CAS), China
Copyright © 2022 Chen, Landis, Wang, Sun, Wang and Wang. This is an open-access article distributed under the terms of the Creative Commons Attribution License (CC BY). The use, distribution or reproduction in other forums is permitted, provided the original author(s) and the copyright owner(s) are credited and that the original publication in this journal is cited, in accordance with accepted academic practice. No use, distribution or reproduction is permitted which does not comply with these terms.
*Correspondence: Hua-Feng Wang, 279745044@qq.com