- 1Gulf Coast Research and Education Center, Institute of Food and Agricultural Sciences, University of Florida, Wimauma, FL, United States
- 2University of Florida, Horticultural Sciences Department, Institute of Food and Agricultural Sciences, Gainesville, FL, United States
- 3University of Florida, Agronomy Department, Institute of Food and Agricultural Sciences, Gainesville, FL, United States
The cultivated strawberry, Fragaria ×ananassa, is a recently domesticated fruit species of economic interest worldwide. As such, there is significant interest in continuous varietal improvement. Genomics-assisted improvement, including the use of DNA markers and genomic selection have facilitated significant improvements of numerous key traits during strawberry breeding. CRISPR/Cas-mediated genome editing allows targeted mutations and precision nucleotide substitutions in the target genome, revolutionizing functional genomics and crop improvement. Genome editing is beginning to gain traction in the more challenging polyploid crops, including allo-octoploid strawberry. The release of high-quality reference genomes and comprehensive subgenome-specific genotyping and gene expression profiling data in octoploid strawberry will lead to a surge in trait discovery and modification by using CRISPR/Cas. Genome editing has already been successfully applied for modification of several strawberry genes, including anthocyanin content, fruit firmness and tolerance to post-harvest disease. However, reports on many other important breeding characteristics associated with fruit quality and production are still lacking, indicating a need for streamlined genome editing approaches and tools in Fragaria ×ananassa. In this review, we present an overview of the latest advancements in knowledge and breeding efforts involving CRISPR/Cas genome editing for the enhancement of strawberry varieties. Furthermore, we explore potential applications of this technology for improving other Rosaceous plant species.
1 Introduction
The cultivated strawberry (Fragaria ×ananassa) is among the most economically important fruit crops in the world. According to the Food and Agricultural Organization of the United Nations, during 2021, more than nine million tonnes of strawberries were produced from 389,665 ha around the world (FAO, 2023). The cultivated strawberry as it is today resulted from a series of interspecific hybridizations, with the final cross between octoploid progenitors occurring only approximately 300 years ago (Edger et al., 2019; Whitaker et al., 2020). Evolutionary analysis of Fragaria ×ananassa suggested genomic contributions of four diploid progenitor species: Fragaria vesca, Fragaria iinumae, Fragaria viridis, and Fragaria nipponica (Edger et al., 2019; 2020). However, analysis from other groups has suggested that both F. viridis and F. nipponica are not among the diploid progenitors (Liston et al., 2020; Jin et al., 2023; Lyu et al., 2023; Session and Rokhsar, 2023). As identification of the progenitor species may enable greater prediction of polyploid responses to environmental stress and climate change (Liston et al., 2020), further analysis of the evolutionary history of strawberry will be necessary to confirm the identities of the diploid progenitor species.
Fragaria ×ananassa is a highly heterozygous, allo-octoploid species (2n = 8x = 56) with a phased genome length of approximately 780 Mb (Hardigan et al., 2021a; Han et al., 2022). Due to the complex nature of the allo-octoploid genome and high genomic heterozygosity, trait discovery and gene functionalization studies in strawberry are commonly performed in the diploid woodland strawberry, Fragaria vesca. Fragaria vesca is used as a model system for strawberry research for several reasons: it has a small genome size of approximately 240Mb, has a short life cycle, is relatively responsive to transformation, and is easy to propagate using both seeds and runner cuttings (Oosumi et al., 2006). Fragaria vesca is additionally the closest relative of the transcriptionally dominant diploid subgenome of Fragaria ×ananassa (Hardigan et al., 2021b), further lending to its value as a model system for strawberry research. While trait discovery and gene functional studies performed in Fragaria vesca are useful for inferring mechanisms of commercially valuable traits in the cultivated strawberry, there are also drawbacks to relying on the diploid system. The largest drawback to working with the diploid strawberry is that Fragaria vesca tends to be more homozygous at a given locus as compared to Fragaria ×ananassa. Martín-Pizarro et al. (2019) identified one such instance of this when they identified at least five alleles of the FaTM6 locus across the four homoeologous chromosomes of octoploid strawberry and only a single homozygous FvTM6 locus in the diploid strawberry. Due to the differences in heterozygosity, it can be difficult to determine which allele(s) in Fragaria ×ananassa contribute to a phenotype using data from Fragaria vesca alone, hindering attempts at genomics-assisted improvement of commercially relevant varieties.
Recent advancements in genome sequencing technology and gene annotation have enabled the assembly of numerous strawberry genomes encompassing a range of ploidy levels (Qiao et al., 2021; Han et al., 2022; Song et al., 2023a). Enabled by these genome assemblies, CRISPR/Cas genome editing is increasingly applied for the study and improvement of key strawberry traits. While not currently widespread due to several challenges, genome editing in allo-octoploid strawberry offers a unique opportunity to precisely modify traits of interest. Alternatively, tools such as RNAi, stable or transient overexpression of transgenes have been utilized to validate gene function and support identification of candidate genes. Plant breeding has also made use of genomics-assisted techniques such as marker-assisted selection and genomic selection for varietal improvement, enabled by new methods for QTL discovery and analysis.
This review covers the genomic tools and technologies available for genomics-assisted improvement of cultivated strawberries, in addition to multi-omics technologies and their applications in identifying candidate QTLs and genes. Reports of successful CRISPR/Cas genome editing for traits associated with strawberry fruit quality and production are discussed herein. In addition to these reports, this review suggests additional candidates for genome editing based on analyses in strawberry and other crops.
2 Advanced genomic resources in strawberry
Genomic complexity of the polyploid genome is one of the major challenges of genome editing in octoploid strawberry. The first high quality, chromosome-scale reference genome of Fragaria ×ananassa cv. ‘Camarosa’ was developed in 2019 (Edger et al., 2019). Before the release of the first octoploid strawberry genome, there were few genome assemblies available for polyploid species, as the homoeologous nature of the subgenomes made assembly of sequences difficult (Wang et al., 2023b). As such, molecular genetic analysis of Fragaria ×ananassa prior to 2019 relied on early genome assemblies of Fragaria vesca, the first of which was published in 2011 (Shulaev et al., 2011). Recent rapid advancements in genome sequencing technology have resulted in the release of additional annotated octoploid genome sequences of increasing quality (Table 1) (Wang et al., 2023b). Advancements in genome sequencing in strawberry have not been limited only to the octoploid, as new high-quality genomes have also recently been released for the diploid model species, Fragaria vesca (Alger et al., 2021; Joldersma et al., 2022). As improvements to genome sequencing technology continue, it will be possible to better understand genomic complexity in not only octoploid strawberry, but in other polyploids as well.
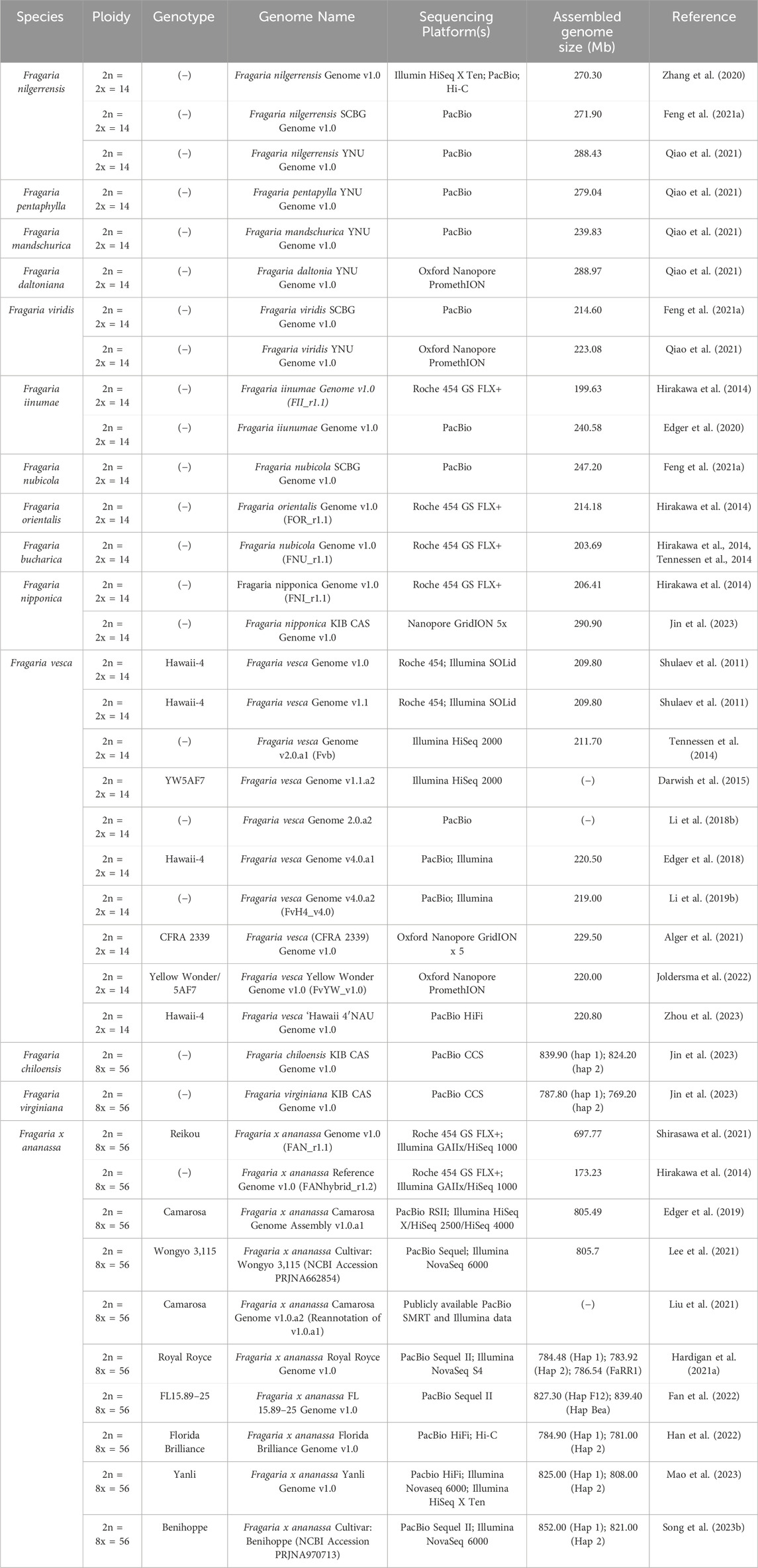
Table 1. Compilation of publicly available genome assemblies for various Fragaria species. (−) indicates value not reported.
Recently, multiple single nucleotide polymorphism (SNP) arrays for octoploid strawberry have been developed to assist with quantitative trait loci (QTL) discovery, including 50K and 90K arrays (Bassil et al., 2015; Verma et al., 2017). Using these tools to identify SNPs correlated to QTLs enables the development of molecular markers which can be utilized in breeding (Jung et al., 2017). Marker assisted selection (MAS) is a method of precision genomics-assisted breeding which relies on the implementation of genetic markers and trait associations to inform selection (Collard and Mackill, 2008). Large numbers of marker-trait associations have been generated through QTL mapping studies (Collard and Mackill, 2008; Rey-Serra et al., 2021) which makes MAS a powerful tool for precision breeding. Implementation of high-throughput assays such as high-resolution melting (HRM) and simple sequence repeat analysis (SSR) paired with DNA markers and rapid high-throughput DNA extraction methods have enabled rapid improvement of octoploid strawberry varieties at the University of Florida (Noh et al., 2017). This success highlights the significant potential of MAS for varietal improvement of fruit crops.
Genomic selection (GS) is a method of selection which utilizes genome-wide variation and phenotypic data to predict phenotypes of an unobserved population (Bernardo, 1994; Meuwissen et al., 2001; Goddard and Hayes, 2007; Montesinos-López et al., 2021). GS offers the potential for increased genetic gain within a breeding program, as it enables increased selection intensity, selection accuracy, and reduction of the generational interval (Werner et al., 2023). Additionally, GS enables faster selection of clonally propagated crops such as those in the Rosaceae family by predicting their performance as clones while they are still in the seedling stage, allowing for earlier analysis of traits that would otherwise require further physiological development, such as fruit flavor and shelf life (Werner et al., 2023). Due to the complexity of the polyploid genome, generation of genome-wide SNP arrays can be complicated, contributing to the delay in adoption of GS methods for breeding of polyploid crops (Zingaretti et al., 2019). Despite the challenges posed by genomic complexity of polyploids, the continuous release of high-quality reference genomes has allowed for the parallel improvement of SNP genotyping methods. To aid in adoption of GS in polyploids, software such as polyploid Sequence Based Virtual Breeding (pSBVB) has been developed to simulate and evaluate GS strategies in polyploids and is equipped to simulate differences between allo- and autopolyploids (Zingaretti et al., 2019). Numerous reports have now been made for successful application of GS in octoploid strawberry (Gezan et al., 2017; Whitaker et al., 2017; Osorio et al., 2021). In a study by Yamamoto et al. (2021), 105 inbred strawberry lines were developed and used to train a GS model based on phenotyping data for petiole length, leaf area, Brix, fruit firmness, and pericarp color. Using the model to predict phenotypic values for a F1 hybrid testing population derived from these 105 lines revealed that phenotypic data collected from the parental inbred lines was sufficient to predict the F1 hybrid phenotypes when the model accuracy in cross-validation is sufficient (Yamamoto et al., 2021). Pincot et al. (2020) applied GS to strawberry to evaluate improve Verticillium wilt resistance. While the inclusion of wild genotypes in the training population reduced accuracy, the results suggested a strong potential for GS to identify superior resistant individuals if the model was sufficiently trained (Pincot et al., 2020).
Due to the wide range of research in strawberry, the volume of available genomic resources has increased significantly. As such, the Genome Database for Rosaceae (GDR) was created to house a wide range of tools and data. Among these tools is a compendium of strawberry DNA tests which can be downloaded from GDR1 and implemented in breeding programs (Oh et al., 2019).
2.1 Multi-omics approaches for trait discovery for improving strawberry
Enabled by the release of high-quality genome assemblies, genomic approaches have been applied to help identify candidate genomic regions and genes for several key strawberry traits, including flavor (Oh et al., 2021; Fan et al., 2022; 2023), disease resistance (Mangandi et al., 2017; Nellist et al., 2019; Nelson et al., 2021; Salinas et al., 2019), fruit firmness (Lee et al., 2021), fruit quality (Verma et al., 2017), and fruit shape (Nagamatsu et al., 2021). As genomic technologies continue to improve, they will allow for greater understanding of the genetic interactions and mechanisms underlying traits of breeding interest. QTL mapping has been performed in strawberry to identify loci underlying major quality and production traits such as day-neutrality, runner production, disease resistance, and fruit quality traits, among many others (Weebadde et al., 2008; Castro et al., 2015; Cockerton et al., 2018; Hossain et al., 2019; Alarfaj et al., 2021). Similarly, genome-wide association studies (GWAS) have been applied in strawberry for the discovery of numerous major traits (Pincot et al., 2018; Wada et al., 2020; Saiga et al., 2022). Recent advancements in genome sequencing technology have also enabled the analysis of large populations and generation of pangenomes, from which structural variants associated with key traits can be identified (Bohra et al., 2020). Pangenomics has been applied in strawberry to identify patterns in fruit color (Qiao et al., 2021). Analysis of strawberry pangenomes also resulted in interesting findings about strawberry evolution and domestication. Based on transposable element analysis of a pangenome constructed from 10 high-quality strawberry genomes, Lyu et al. (2023) suggested that Fragaria viridis may not be one of the diploid Fragaria ×ananassa ancestors as previously thought. Additionally, Qiao et al. (2021) discovered a new diploid strawberry species during assembly of their own pangenome. Discovery of transposable elements and other structural variants can be difficult when performing analysis of single genomes, however, such variants have been known to impact major agronomic traits (Tao et al., 2019), and are easier to identify through pangenome analysis. Thus, continued exploration of strawberry pangenomes may yield further insight into other major fruit quality and production traits and may assist in unravelling the complex evolutionary history of cultivated strawberry. Genomics-assisted technologies have also been widely implemented for trait discovery in crops outside of Rosaceae. New methods of QTL mapping have demonstrated capacity to resolve QTL candidates within a window of only a few kilobases (Bohra, 2013; Varshney et al., 2014; Zhang et al., 2019; Bohra et al., 2020), and in some cases, were able to generate QTL regions of which the resolution is comparable to the outcomes of sequence-based GWAS (Zhang et al., 2019; Bohra et al., 2020). Implementation of these new techniques in strawberry may enable further trait discovery for key agronomic traits.
In addition to the application of genomics for candidate gene identification, transcriptomics has been applied in strawberry to map expression quantitative trait loci (eQTLs) related to various fruit traits, including flavor (Sánchez-Sevilla et al., 2014; Barbey et al., 2020; 2021; Fan et al., 2022) disease resistance (Barbey et al., 2019), ripening and softening, and several others (Barbey et al., 2020). Strawberry transcriptome data has also recently been used to explore major postharvest issues, such as host responses to Botrytis cinerea infection (Yu et al., 2021) and differences in shelf life between cultivars (Min et al., 2020). In both cases, several candidate genes were identified which may be involved in plant defense, regulation of senescence, and shelf life (Min et al., 2020; Yu et al., 2021). Transcriptomic analyses of strawberry resulted in updates to existing genome annotations (Liu et al., 2021) and new transcriptome assemblies (Sánchez-Sevilla et al., 2017). Sufficient strawberry transcriptomic data has even been generated to allow for a meta-analysis of fruit ripening which resulted in the identification of previously unrevealed differentially expressed genes (DEGs) (Yi et al., 2021). Additionally, it is possible to identify allelic contributions based on relative expression patterns using transcriptomic data (Castillejo et al., 2020; Chandra et al., 2021; Oh et al., 2021). Considering this, transcriptomics could be a powerful tool for identifying candidates in polyploid crops such as strawberry, where unraveling the allelic contribution to a specific trait across homoeologous chromosomes remains challenging. As such, identification of transcriptionally dominant alleles may inform targeted trait improvement.
Despite the advancements in genome sequencing and transcriptomics which facilitate genome editing in octoploid strawberry, gene functional studies still rely heavily on transgenic approaches including RNA interference (RNAi) and overexpression (OE). Numerous traits, including several of postharvest interest, have been explored through transient and transgenic approaches in strawberry (Table 2). RNAi is an efficient tool for validating gene function through post-transcriptional gene silencing induced by double stranded RNA (Singh and Roychoudhury, 2023). RNAi and antisense approaches have also informed successful CRISPR/Cas strategies in strawberry (García-Gago et al., 2009; Paniagua et al., 2020; Paniagua et al., 2022; López-Casado et al., 2023). Transgenic application of tools like RNAi, antisense downregulation, and OE can be used to identify candidate genes prior to genome editing. Such transgenic lines depend on the continued expression of the recombinant constructs, which can vary depending on environmental or developmental effects. As such, these transgenic lines are not ideal to facilitate varietal improvement. Instead, identification and functionalization of candidate genes can be used to inform subsequent CRISPR/Cas genome editing. With the correct strategy, candidate genes can be knocked in or out, and alleles can be swapped for superior versions, allowing for altered gene expression or function and resulting phenotypes. The corresponding mutants can then be used in breeding schemes to further improve elite varieties. As such, transgenic approaches can be important tools to inform genome editing strategies for improvement of strawberry.
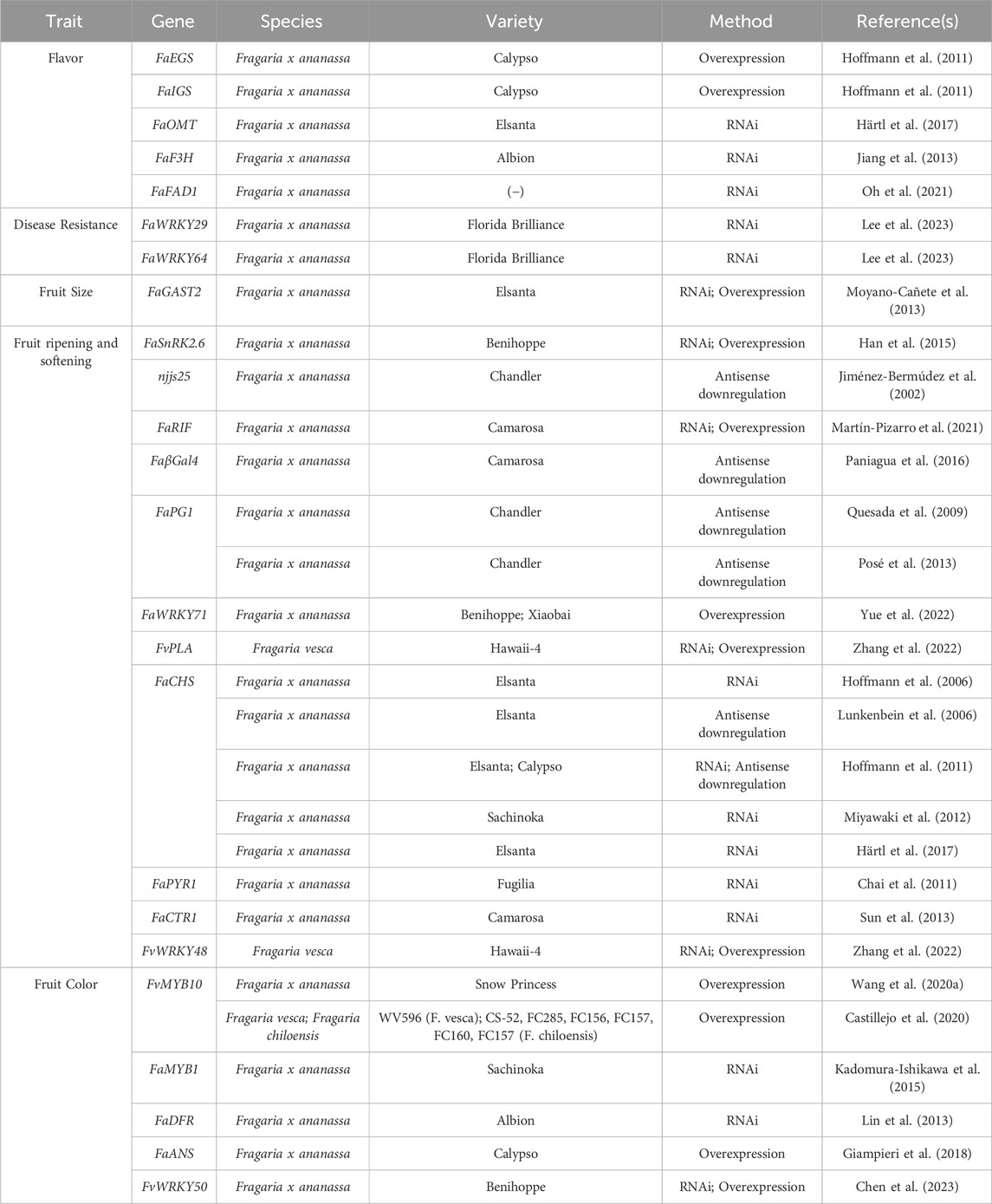
Table 2. Reported applications of transgenic approaches in strawberry for analysis of fruit quality and postharvest traits. (−) indicates value not reported.
Since metabolites are major contributors to fruit flavor and quality, it is necessary to identify genes associated with their production. Metabolomics can supplement transcriptomic and genomic data to support gene discovery on a biochemical level. In strawberry, metabolomics is frequently used in flavor studies (Schwab et al., 2008; Chambers et al., 2014; Fan et al., 2022), however, metabolomics has also been implemented in studies of plant stress response (Antunes et al., 2019), fruit development and ripening (Vallarino et al., 2018), and response to blue light (Chen et al., 2020). Like metabolomics, proteomics can be used in tandem with transcriptomic and genomic data to facilitate gene discovery. Application of proteomics and transcriptomics has been used to analyze postharvest quality changes during storage under different conditions, including controlled ozone treatments (Chen et al., 2019) and temperature stress (Lv et al., 2022). In both cases, comparisons of the differentially expressed proteins (DEPs) with the expression patterns of their respective genes found that proteosome responses mimicked the changes in postharvest quality, further supporting their proposed roles in stress response (Chen et al., 2019; Lv et al., 2022).
Multi-omics has been employed for trait discovery in strawberry and other crop species. In tomato, the correlation of SNPs, transcripts, and metabolites was used to identify new genes and pathways dictating major fruit traits (Zhu et al., 2018; Gaston et al., 2020). Similar application of multi-omics analysis in strawberry may discover novel pathways and gene candidates supporting fruit quality and production. If diverse evidence points to major genes for a desirable trait, CRISPR/Cas-mediated editing of the major gene or its repressor should have a high potential for crop improvement. As such, continual integration of genomics, transcriptomics, metabolomics, and proteomics is critical to inform CRISPR/Cas9 approaches for improvement of strawberry.
3 Genetic transformation and regeneration of strawberry
Following trait discovery, genetic transformation can be performed to validate candidate gene function (Figure 1). While CRISPR/Cas-mediated genome editing has yet to be widely implemented and optimized for Fragaria species, numerous reports of transgenic modifications have been made. Agrobacterium-mediated transformation is currently the most applied transformation method for strawberry. Protocols using agrobacterium have undergone significant optimization to improve transformation efficiency. Thus far, protocols have been established for Agrobacterium-mediated transformation and subsequent regeneration of a range of tissues (Li et al., 2018a; Feng et al., 2019; Feng et al., 2021b; Wilson et al., 2019; Duan et al., 2021; Mao et al., 2022; Yan et al., 2023). In strawberry, agrobacterium-mediated transformation most commonly uses Agrobacterium tumefaciens strains LBA4404, GV3101, and MP90. Leaves are the most common explant material (Table 3). Transient methods of agrobacterium-mediated transformation have also been developed for fruit (Carvalho et al., 2016; Dai et al., 2020; Zeng et al., 2021; Mao et al., 2022; Lee et al., 2023) to study a range of mechanisms and traits, as well as to analyze the performance of DNA constructs prior to stable transformation. Particle bombardment (Agius et al., 2005) and protoplast transfection (Gou et al., 2020) have also been applied in strawberry for transient analyses. However, despite the demonstrated success of both methods in such analyses, stable transformation of strawberry with these approaches remains a challenge.
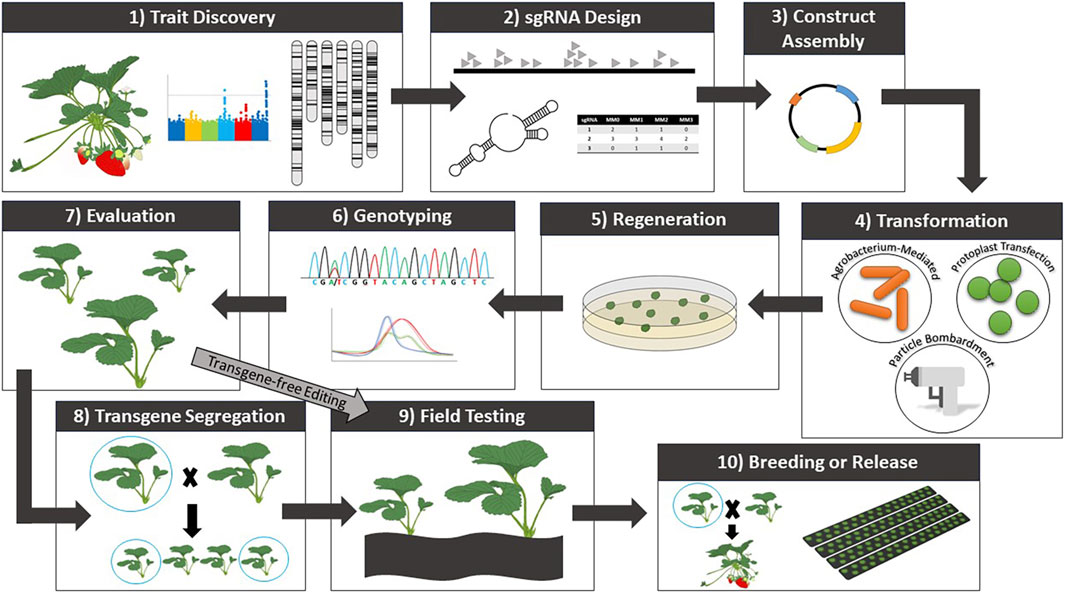
Figure 1. General workflow for trait discovery and CRISPR/Cas-mediated genome editing in strawberry.
Transformation of strawberry has also been performed using the hairy root system (Yan et al., 2023). Hairy roots are valuable for functional analysis of root traits as well as for validation of transgenic and genome editing methods due to the relatively short period of root development (Ozyigit et al., 2013). Several advancements have been made to Agrobacterium-mediated transformation technologies, including improved ternary systems (Anand et al., 2018), auxotrophy of various amino acids (Aliu et al., 2020; Prías-Blanco et al., 2022), and use of a CRISPR RNA-guided integrase system (Vo et al., 2021; Aliu et al., 2022), however, there has been little application of these advanced systems in strawberry (Oosumi et al., 2006).
Some transgene-free methods, such as ribonucleoprotein (RNP) bombardment and RNP transfection of protoplasts, have not been reported in strawberry but have demonstrated success in a range of crops (Liu et al., 2020; Zhang et al., 2021a; Najafi et al., 2023). Other methods of transformation and editing, such as RNA bombardment (Li et al., 2019a) and virus-mediated transformation (Tian et al., 2015) have been sporadically applied in strawberry, indicating a need for further development. Transgene-free targeted mutagenesis approaches for varietal development fall within improved consumer acceptance and reduced regulatory constraints. Thus, continued refinements of genomic tools, transformation and genome editing strategies will position CRISPR/Cas technology as primary tool for gene function validation and crop improvement.
Further development of efficient protoplast isolation, transformation, and regeneration is also important for future transgene-free editing of strawberry. Isolation and regeneration of protoplasts is well established for strawberry (Nyman and Wallin, 1988; 1992a; Barceló et al., 2019; Gou et al., 2020). However, while protocols have been established for transient analyses in protoplasts, few reports of successful regeneration involve transformed materials (Nyman and Wallin, 1992b; Pattanaik et al., 2004; Gou et al., 2020). As such, it is necessary to continue developing methods to transform and regenerate plants from strawberry protoplasts as a foundation for transgene-free genome editing.
As has been established in other species, transformation and regeneration of strawberry depends on several factors, and protocol optimization can be challenging. Experiments to optimize strawberry transformation have observed a wide range of transformation and regeneration efficiencies which vary significantly between genotypes (Zakaria et al., 2014). Other factors, such as transformation method and explant material, have also been reported to impact regeneration efficiency, and response to these factors also varies strongly by genotype (Table 3). Taken together, these findings suggest that some genotype-specific optimization of protocols will be necessary for efficient genetic transformation. Additionally, transformation and regeneration efficiencies tend to be higher for diploid strawberry than octoploid strawberry, even when other factors are held constant between species (Table 3). As Fragaria ×ananassa is the species of economic interest, continued optimization to improve both transformation and regeneration efficiencies will be essential to facilitate genome editing for varietal improvement.
4 Recent advances and resources in CRISPR/Cas-mediated genome editing in strawberry
CRISPR, or Clustered Regularly Interspaced Palindromic Repeats, is a genome editing system derived from a bacterial defense network. In bacteria, this defense network operates in two phases to incorporate short fragments of invading DNA into the bacterial genome and then use these sequences to recognize and cleave foreign DNA based on the presence of a protospacer adjacent motif (PAM) (Doudna and Charpentier, 2014; Vigouroux and Bikard, 2020). For genome editing via CRISPR/Cas systems, this bacterial defense pathway is manipulated to target specific sequences within a genome of interest. Unlike other methods of genome editing, such as zinc-finger nucleases (ZFNs) and transcription activator-like nucleases (TALENs), which require substantial protein engineering, CRSIPR/Cas genome editing can be performed simply through a change in the single guide RNA (sgRNA) sequence (Doudna and Charpentier, 2014). The ability to switch editing targets quickly without need for protein engineering has played a major role in the rise of popularity of CRISPR/Cas genome engineering. Double stranded breaks (DSBs) are generated when a Cas endonuclease cleaves DNA at a targeted site using a sgRNA as reference (Cong et al., 2013; Mali et al., 2013; Doudna and Charpentier, 2014). These DSBs can then be repaired through non-homologous end joining (NHEJ), or template mediated homology-directed repair (HDR). NHEJ is error prone, often resulting in insertions or deletions that cause loss of gene function (Cong et al., 2013; Mali et al., 2013; Chen et al., 2022). Targeted mutagenesis using NHEJ mediated repair of CRISPR/Cas-mediated DSBs has been reported in many Rosaceous crops, including apple (Malnoy et al., 2016; Pompili et al., 2020), pear (Charrier et al., 2019; Pang et al., 2019), raspberry (Miller, 2019), and strawberry (Martín-Pizarro et al., 2021; López-Casado et al., 2023). In contrast, template mediated HDR allows precise conversion of targeted single nucleotides or insertion of a specific sequence. Allelic variants differing in single-nucleotide polymorphisms often confer improvement of agronomic traits. HDR pathways can be leveraged to replace alleles with superior variants and has successfully been implemented in crops such as maize, rice, and sugarcane (Shi et al., 2017; Wang et al., 2017; Oz et al., 2021). Reports of HDR-mediated gene targeting are still lacking in strawberry, likely due to low efficiency caused by infrequent occurrence of HDR, competition with NHEJ for DSB repair, and inadequate repair template in close proximity to the DSB site (Chen et al., 2022).
Similar to template mediated HDR, both base and prime editing can generate precision nucleotide substitutions in target genes. Base editing occurs as the result of a catalytically impaired Cas nuclease, such as Cas nickase (nCas) or dead Cas (dCas), fused to a nucleotide deaminase (Molla et al., 2021) and results in an irreversible base conversion without the need for DSBs or an exogenous template (Azameti and Dauda, 2021). Base editing requires the use of different deaminases depending on the desired nucleotide substitution, is currently limited to six of the 12 possible base-swaps, and may result in bystander mutations (Molla et al., 2021). In contrast, prime editing, which occurs as the result of fusing a nCas nuclease with a reverse transcriptase, is capable of generating all 12 possible substitutions as well as small indels in exchange for lower editing efficiency (Molla et al., 2021). Base editing has been applied for the creation of precision nucleotide substitutions in strawberry to support the fine tuning of the sugar content of the strawberry fruit (Xing et al., 2020). While prime editing has not been reported in strawberry, it has been successfully applied in tomato, rice, and wheat (Lin et al., 2020; Xing et al., 2023), demonstrating its potential for precision nucleotide substitution in plant systems.
In addition to generating nucleotide substitutions through base or prime editing, CRISPR/Cas can also be applied to modulate gene expression patterns and epigenetic regulation. By fusing dCas with different effector proteins, it is possible to achieve efficient targeted activation (CRISPRa), repression (CRISPRi), or epigenome modifications (Pan et al., 2021).
While genome editing using the CRISPR/Cas system has largely focused on the use of the Cas9 endonuclease, additional Cas nucleases have been engineered to improve the flexibility of the CRISPR genome editing system by relaxing the requirements for a specific protospacer adjacent motif and altering nuclease function (Guilinger et al., 2014; Shen et al., 2014; Trevino and Zhang, 2014; Tsai et al., 2014; Anders et al., 2016; Komor et al., 2016; 2017; Havlicek et al., 2017; Harrington et al., 2018; Liu et al., 2019; Anzalone et al., 2020; Ghogare et al., 2020; Zhang et al., 2021b; Sukegawa et al., 2023). This is particularly useful for generation of precision nucleotide substitutions using base or prime editing (Wang et al., 2020b; Kantor et al., 2020; Mishra et al., 2020; Huang and Puchta, 2021).
Genome editing is a powerful tool for crop improvement, as it allows for precise, targeted mutation in one or few genes without altering the plant’s genetic background. Genome editing can be an efficient method for varietal improvement as co-editing of multiple genes or alleles by multiplex editing allows researchers to accelerate the generation of desired combinations in elite germplasm without undergoing meiotic recombination (El-Mounadi et al., 2020). In contrast, conventional breeding schemes typically require numerous generations and backcrossing to improve gene or allele combinations for a single trait of interest. Genome editing with CRISPR/Cas also enables the introduction of traits that do not exist within a breeding germplasm. For example, there are currently no widely available breeding sources of resistance to Botrytis cinerea in strawberry, and previous breeding efforts to increase resistance to Botrytis fruit rot (BFR) have been ineffective (Petrasch et al., 2019). However, substantial research has been performed to identify susceptibility genes related to BFR and other strawberry diseases which could make for useful knockout targets in the future.
4.1 Challenges for genome editing in octoploid strawberry
While the potential benefits of CRISPR/Cas genome editing in Fragaria ×ananassa are numerous, there are several challenges which must be overcome. Octoploid strawberries are highly heterozygous as compared to diploid strawberries (Martín-Pizarro et al., 2019), which can make target gene identification and design of efficient sgRNAs difficult (May et al., 2023). Additionally, the four homoeologous subgenomes of Fragaria ×ananassa are not separated from each other, rather, they have undergone numerous homoeologous exchanges which resulted in increased genomic complexity (Whitaker et al., 2020).
Traditionally, transgene-free genome edited plants are generated through sexual segregation, which is often a labor-intensive and time-consuming process (Gao, 2021). Cultivated strawberries are asexually propagated hybrids, meaning segregation of transgene-free plants through segregation is often impractical. Instead, it is necessary to continue developing other transgene-free genome editing methods for the improvement of strawberry. In addition to optimizing transformation procedures, further development of systems such as the transgene killer CRISPR (TKC), which is able to automatically self-destruct the transgene through inclusion of suicide genes in the CRISPR/Cas construct (He et al., 2018; He et al., 2019; Gu et al., 2021), may enable transgene-free genome editing of strawberry. To date several studies for transgene-free genome editing of Rosaceous crops have been performed (Malnoy et al., 2016; Osakabe et al., 2018; Pompili et al., 2020), though regeneration of explants and selection of transgene-free plants remains a challenge.
4.2 Target traits for CRISPR genome editing in cultivated strawberry (F. ×ananassa)
Genome editing in strawberry can be divided into two themes; editing performed in the diploid strawberry and editing performed in the octoploid strawberry. Genome editing is more commonly performed in diploid strawberry due to the simple nature of its genome and its status as a model system for Rosaceae. In the diploid strawberry, genome editing has been successfully employed to manipulate numerous traits (Table 4). Until recently, the complexity of the octoploid genome posed a significant challenge to CRISPR/Cas genome editing, and as such, far less exploration of genome editing in Fragaria ×ananassa has been performed. Due to the availability of new, high-quality octoploid genome assemblies, genome editing has recently been applied for the improvement of several traits (Table 4; Figure 2) and may become a powerful tool for trait discovery and gene characterization in cultivated strawberry.
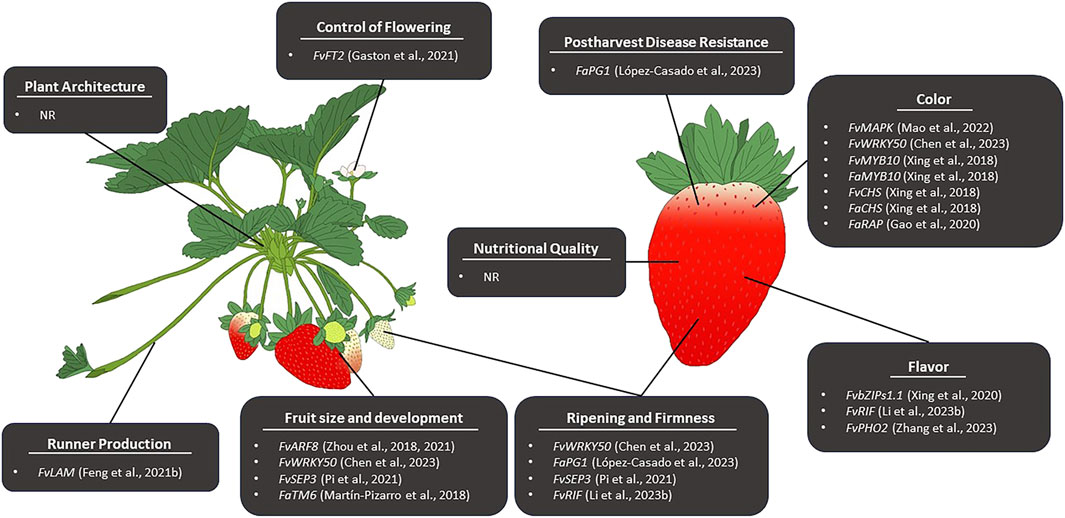
Figure 2. Reported CRISPR/Cas-mediated editing for the improvement of pre- and postharvest traits associated with strawberry fruit quality and production. NR indicates no genome editing has been reported to affect a given trait.
4.2.1 Manipulation of runner production to increase fruit production
In strawberry, differentiation of runners and branch crowns is mutually exclusive and is influenced by a range of environmental factors (Thompson and Guttridge, 1959; Hytönen et al., 2009; Mouhu et al., 2013; Tenreira et al., 2017; Caruana et al., 2018) However, since each plant produces a limited number of axillary meristems, runner production is considered inversely proportional to fruit production (Tenreira et al., 2017). For this reason, runner removal is a common cultural practice and has demonstrated positive impacts on both fruit quality and yield (Sønsteby et al., 2021). Many studies have attempted to characterize the relationship between fruit and runner production through manipulation of environmental conditions and plant hormones (Hartmann, 1947; Thompson and Guttridge, 1959; Mouhu et al., 2013; Qiu et al., 2019).
As genomic resources became more widely available, studies began to focus on the genes which dictate the decision of flowering versus runnering in strawberry. Suppressor of Overexpression of Constans1 (FvSOC1), FvGA20ox4, FvRGA1, and Loss of Axillary Meristems (FvLAM) have all been identified as runner-associated genes (Mouhu et al., 2013; Tenreira et al., 2017; Caruana et al., 2018; Hytönen and Kurokura, 2020; Feng et al., 2021b). All runner-associated genes which have been identified to date are related to gibberellic acid biosynthesis, suggesting that gibberellic acid plays a major role in the decision between flowering and runnering. The full pathway of gibberellic acid biosynthesis in strawberry remains to be elucidated, however the proposed pathway has undergone continuous expansion as new runnering and flowering-associated genes are identified.
Despite the identification of numerous genes associated with runner production, so far CRISPR/Cas9 genome editing has only been reported in FvLAM in Fragaria vesca (Feng et al., 2021b), and no reports of genome editing of runner-associated genes in Fragaria ×ananassa have been made. CRISPR/Cas9 genome editing has also been applied in tomato and potato for the manipulation of similar traits (Zsögön et al., 2018; Cui et al., 2020; Tang et al., 2022; Tuncel and Qi, 2022), indicating that genome editing can be used effectively to alter plant growth habits. Plant architecture has been shown to have major impacts on yield in numerous crops (Sakamoto and Matsuoka, 2004; Srivastava et al., 2019), making the trait a prime candidate for manipulation via genome editing.
4.2.2 Plant architecture and control of flowering
The timing and duration of flowering play pivotal roles in determining yield potential and harvest season length (Koskela et al., 2012). Manipulation of daylength sensitivity can enable earlier harvest (Soyk et al., 2017) and can even lead to multiple harvests within a single season (de Camacaro et al., 2002; Hancock et al., 2008). During the domestication of strawberry, development of perpetual flowering through photoperiod insensitivity was essential for extending both the range and production period (Gaston et al., 2021). Increased branching can also support increased inflorescences, enabling a greater yield per plant (Premsekhar and Rajashree, 2009). Since the axillary meristem-derived branch crowns are the primary bearer of inflorescences in strawberry, it may be possible to increase fruit yield by increasing floral branching.
Terminal Flower1 (FvTFL1), Flowering Locus T (FvFT2 and FvFT3), and FvWRKY50 were identified as flowering-related genes and shown to interact with each other to control photoperiod response and flowering architecture (Iwata et al., 2012; Koskela et al., 2012; Mouhu et al., 2013; Gaston et al., 2021; Chen et al., 2023). While timing, duration, and development of flowers are important considerations for fruit production, reports of successful editing have been limited to diploid strawberry. FvFT2 and FvWRKY50 have undergone successful genome editing (Gaston et al., 2021), however, editing of other strawberry flowering-related genes has not been reported. Several genes have been identified in other crops with respect to plant architecture and flowering. Branched 1 (AtBRC1), Branched 2 (AtBRC2), SlBRC1b, AtMAX1, AtMAX2, AtCYP79, and AtSPS have been identified to negatively affect branching (Reintanz et al., 2001; Tantikanjana et al., 2001; Stirnberg et al., 2002; Greb et al., 2003; Aguilar-Martínez et al., 2007; Finlayson, 2007; Martín-Trillo et al., 2011). Similarly, Self Pruning 5g (SlSP5G) and Flowering Locus C (BrFLC2) resulted in early flowering and daylight insensitivity (Soyk et al., 2017; Jeong et al., 2019). As no editing of plant architecture or flowering-associated genes has been reported in octoploid strawberry, further functional analysis of known genes is necessary for the improvement of existing varieties.
4.2.3 Fruit development
Further study of fruit development genes in strawberry may also enable improvement of fruit production. Parthenocarpy may enable more consistent yields under variable conditions (Hu et al., 2023), and larger fruits tend to be easier to harvest and more desirable to consumers (Hortyński et al., 1991). Both auxin and gibberellic acid have been well established as major regulators of strawberry fruit development (Sharma and Singh, 2009; Liu et al., 2012; Kang et al., 2013; Feng et al., 2019; Fuentes et al., 2019; Zhao et al., 2021) though morphological and environmental factors are also major contributors (Hortyński et al., 1991; Mackenzie et al., 2011; Menzel, 2019; 2021). Through domestication, the average strawberry fruit weight has increased from 1 to 3 g (Fragaria virginiana) to more than 20 g (Fragaria ×ananassa) (Chandler et al., 2012).
Knockout of FvYUC10 altered patterns of auxin accumulation in fruit but resulted in no obvious morphological changes (Feng et al., 2019). RNAi of FvYUC6 was found to negatively affect fruit development (Liu et al., 2014). Other YUC family genes have been identified through transcriptomic studies of strawberry fruit development (Liu et al., 2012; Feng et al., 2019) but have not undergone additional functionalization. Mutations in FvRGA1 and genome editing in Sepallata 3 (FvSEP3) resulted in parthenocarpic fruit development. Genome editing in FvARF8 resulted in increased fruit sizes (Pi et al., 2021; Zhou et al., 2021) and RNAi of FvYUC6 and FveCYP707A4a resulted in reduced fruit sizes (Liu et al., 2014; Liao et al., 2018). Reduction of fruit size was also accomplished through overexpression of Gibberellin Stimulated Transcript (FaGAST1 and FaGAST2) (de la Fuente et al., 2006; Moyano-Cañete et al., 2013). Knockout of Tomato MADS box gene6 (TM6) in both diploid and octoploid strawberry resulted in defects in the anthers and arrested development of the receptacle (Martín-Pizarro et al., 2019), indicating that TM6 also plays a major role in development of both strawberry flowers and fruits.
Significant work has also been performed to explore the mechanisms underlying fruit development in other crops. In apple, a strong QTL for fruit weight was linked to Auxin Response Factor 106 (MdARF106) (Devoghalaere et al., 2012). Silencing of SlIAA7 in tomato resulted in thicker pericarp tissue, and thus, larger fruits (Su et al., 2014). Simultaneous knockout of SlARF8a and SlARF8b resulted in parthenocarpic fruit development and increased parthenocarpic fruit sizes (Hu et al., 2023), and knockout of Fasciated (SlFAS/SlCLV3), Fruit Weight 2.2 (SlFW2.2), and Excessive Number of Floral Organs (SlENO) resulted in increased locule numbers and larger fruits (Zsögön et al., 2018; Yuste-Lisbona et al., 2020). In addition to these genes, some studies have explored the impacts of hormone pathways on fruit development. These studies found that application of gibberellic acid at low concentrations positively impacted speed of development and yield, and decreased production of malformed fruits (Turner, 1963; Sharma and Singh, 2009; Jamal Uddin et al., 2012), further supporting the potential to improve fruit production through manipulation of hormone biosynthesis and signaling.
4.2.4 Fruit flavor
Strawberry aroma is the result of a complex mixture of more than 360 volatile compounds (Zabetakis and Holden, 1997; Zorrilla-Fontanesi et al., 2012; Liu et al., 2023), however, only around six odor-active compounds which significantly contribute to flavor have been identified in cultivated strawberry (Raab et al., 2006; Ulrich et al., 2007; Hoffmann et al., 2011). As in many other fruit species, early breeding efforts in strawberry focused on improving firmness and other morphological traits at the expense of flavor and aroma (Hoffmann et al., 2011). As such, new efforts are underway to improve strawberry flavor and aroma.
Several genes associated with strawberry flavor and sugar content have been identified through QTL mapping, genome-wide association studies, and transcriptomic studies (Raab et al., 2006; Chambers et al., 2012; Zorrilla-Fontanesi et al., 2012; Shanmugam et al., 2017; Jiu et al., 2018; Lee et al., 2018; Porter et al., 2023). RNAi of strawberry Chalcone Synthase (FaCHS) paired with overexpression of either Eugenol Synthase (FaEGS) or Isoeugenol Synthase (FaIGS) resulted in partial restoration of wild strawberry aroma (Hoffmann et al., 2011). Additionally, RNAi of Anthranilic Acid Methyl Transferase (FaAAMT), Anthranilate Synthase Alpha Subunit 1 (FaASa1), FaFAD1, and FaTM9 resulted in changes in volatile profiles and soluble solids content (Pillet et al., 2017; Vallarino et al., 2019; Oh et al., 2021; Fan et al., 2022). Overexpression of FaOMT resulted in increased levels of mesifurane, another key volatile compound in strawberry (Fan et al., 2022). Base editing has also been successfully applied to modify fruit sugar content. Xing et al. (2020) used the A3A-PBE base editor to target the conserved sucrose control uORF of FvbZIPs1.1, resulting in 35 novel genotypes that displayed a range of sugar contents.
While flavor is a primary focus of varietal improvement in cultivated strawberry, there are no reports of successful editing of flavor genes to date. However, CRISPR/Cas has been applied in several fruit crops for flavor improvement. Knockout of SlINVIVH1 and SlVPE5 in tomato resulted in increases in sugar content and total soluble solids content in single and double mutant lines (Wang et al., 2021a). Genome editing has also been applied for the improvement of flavor traits in vegetables. Karlson et al. (2022) reported successful application of CRISPR/Cas12a to reduce pungency in Brassica juncea, resulting in increased consumer appeal without reducing nutritional content. This work was performed within the company Pairwise, in Durham, North Carolina, and salad mixes composed of the edited Brassica juncea plants have recently been commercially released (Brown, 2023), indicating commercial potential for genome edited crops with improved flavor traits. These successes highlight the potential applications of genome editing technology for the improvement of flavor in strawberry.
4.2.5 Fruit color
Consumer preferences of fruit color can vary significantly across the globe (Whitaker et al., 2020), and as such, strawberries are available in a wide range of colors. Strawberry coloration is primarily due to variation in accumulation of anthocyanin in the receptacle and achenes during ripening. As fruit color is an important fruit quality trait for consumers, it is a common focus of selection in breeding programs and has undergone substantial investigation to identify associated genes.
Natural mutations in FaMYB10 have been reported as the only natural sources of color variation in strawberry (Castillejo et al., 2020). These findings are supported by those of others, which have identified significant roles of MYB10 and other MYB family genes in controlling anthocyanin accumulation and biosynthesis in strawberry (Whitaker et al., 2020; Denoyes et al., 2023). However, other genes have been reported to impact fruit color in addition to MYB10. Overexpression of FvMYB10 and Reduced Anthocyanins in Petioles (FvRAP) resulted in restoration of anthocyanin biosynthesis in white fruits (Castillejo et al., 2020; Gao et al., 2020). While there is agreement that FvRAP plays a role in regulation of anthocyanin accumulation in addition to FvMYB10, conflicting conclusions have been reached regarding the position of FvRAP in the pathway. Luo et al. (2018) suggested that FvRAP operates downstream of and may be regulated by FvMYB10, whereas Gao et al. (2020) suggested that FvRAP may participate in a color development mechanism separate from FvMYB10. Xing et al. (2018) attempted CRISPR/Cas9 genome editing of FvMYB10 and FvCHS in strawberry through an agroinfiltration of diploid and octoploid fruits but observed no noticeable delay in anthocyanin accumulation in octoploid fruits and only partial delay in diploid fruits. Knockout of FvWRKY50 resulted in downregulation of several anthocyanin-associated genes, including FvMYB10, in addition to delayed anthocyanin accumulation (Chen et al., 2023), and knockout of FvMAPK3 resulted in similar rates of anthocyanin accumulation but higher total anthocyanin content than empty vector controls (Mao et al., 2022).
Genome editing for fruit color modification has also been implemented in other species. In tomato, CRISPR/Cas9-mediated genome editing was used to generate tomatoes that were yellow, pink, and purple in color (Čermák et al., 2015; Filler Hayut et al., 2017; Deng et al., 2018), indicating the potential to fine tune fruit color through the application of genome editing. This may enable a greater range of fruit color options and greater flexibility to cater to consumer preferences around the world.
4.2.6 Nutritional content
Strawberries have a diverse nutritional composition with high levels of biological compounds and phytochemicals (Giampieri et al., 2012; 2013; 2014; Afrin et al., 2016). Strawberries have also been studied for their clinical effects (Afrin et al., 2016). Pigments often add to both nutritive value and antioxidant content (Kapoor et al., 2022). Despite the role of nutritional quality in strawberry popularity, genes underlying nutritional content mechanisms are not widely studied. However, recently, some groups have begun to focus on methods to increase nutritional quality. Integration of wild genotypes into a breeding germplasm has been shown to facilitate improvements in fruit nutritional content (Diamanti et al., 2012; Diamanti et al., 2014). While studies have identified genetic components underlying differences in nutritional quality but have not reported candidate genes or loci (Capocasa et al., 2008; Tulipani et al., 2008), transcriptomic and metabolomic analysis of strawberry development and ripening identified numerous genes associated with the flavonoid pathway, including several associated with ellagitannins and anthocyanins (Baldi et al., 2018).
Application of biotechnology in the improvement of crop nutritional quality has occurred in several other crops and may help to guide future nutritional improvement of strawberry fruit. Overexpression of AtGalUR resulted in increased vitamin C content in Arabidopsis thaliana, and vitamin C levels in strawberry were found to correlate with expression of the native AtGalUR ortholog, indicating the potential to enhance vitamin C content in cultivated strawberry (Agius et al., 2003). Additionally, knockout of Lycopene Beta Cyclase (SlCYCB) resulted in dark red fruits as result of increased lycopene accumulation (Zsögön et al., 2018). Transgenic insertion of Narcissus pseudonarcissus Phytoene Synthase (NpPSY) and Erwinia uredovora Carotene Desaturase (EuCRTI) resulted in β-carotene production in rice (Paine et al., 2005). PSY and Orange (OR) have additionally been identified as key proteins in carotenoid biosynthesis in Arabidopsis thaliana (Zhou et al., 2015). Several other carotenoid biosynthesis genes have also been identified in strawberry (Zhu et al., 2015), which may be useful candidates for further investigation of increasing nutritional quality.
4.2.7 Fruit ripening and firmness
Strawberries are non-climacteric, meaning they will continue to redden and soften after harvest, but their flavor will not improve (Azam et al., 2019). In strawberry and other non-climacteric fruits, abscisic acid has demonstrated strong impacts on ripening and softening processes (Li et al., 2011). The popular red “fruit” of strawberry is not a true fruit; it is instead an accessory fruit which is derived from an organ known as the receptacle, a modified stem tip (Hollender et al., 2012; Zhou et al., 2021). The true fruit of a strawberry plant are called achenes, and are the small structures located on the surface of the receptacle (Hollender et al., 2012) which consumers commonly mistake for seeds. Despite this, strawberries are typically used as a model system to study ripening, as plants are small, easy to propagate, have a short vegetative phase, and undergo rapid development and ripening (Perkins-Veazie, 1995; Symons et al., 2012). In this review, ripening is considered the parallel processes of color change and softening. Firmness, while directly associated with the ripening process, differs between genotypes at peak maturity and has a significant impact on postharvest handling and shelf-life.
Numerous genes associated with ripening and softening have been identified and functionalized in both diploid and octoploid strawberry. Downregulation and knockout of Ripening Inducing Factor (FaRIF) resulted in delayed ripening of both the receptacle and achenes (Martín-Pizarro et al., 2021; Li et al., 2023b). Sucrose Nonfermenting1-Related Protein (FaSnRK2.6) and Brap2 Ring ZnF UBP Domain-Containing Protein (FaBRIZ) were found to promote ripening when silenced (Han et al., 2015; Wang et al., 2023a), while downregulation of Polygalacturonase 1 (FaPG1), a β-galactosidase gene (FaβGAL4), and a pectate lyase gene (Fanjjs25) resulted in increased firmness and reduced postharvest softening (Jiménez-Bermúdez et al., 2002; Garcia-Gago et al., 2009; Quesada et al., 2009; Posé et al., 2013; Paniagua et al., 2016; Paniagua et al., 2020; Paniagua et al., 2022). Knockout of FaPG1 and FvSEP3 resulted in significant increase in firmness and reduced postharvest softening, and delayed ripening, respectively (Pi et al., 2021; López-Casado et al., 2023). Several members of the WRKY transcription factor (TF) family have also been implicated in strawberry ripening. Overexpression of FaWRKY71 resulted in increased anthocyanin content and expression of softening-related enzymes (Yue et al., 2022), and transgenic lines for FvWRKY48-RNAi displayed significant delays in both fruit development and ripening, as well as increased fruit firmness (Zhang et al., 2022). Lastly, knockout of FvWRKY50 resulted in delayed anthocyanin accumulation and ripening, though effects on fruit firmness and softening were not reported (Chen et al., 2023). In tomato, knockout of Pectate Lyase (SlPL) resulted in firmer fruits, and knockout of Polygalacturonase 2a (SlPG2a) and β-Galactanase (SlTBG4) resulted in a decrease in pericarp color index (Wang et al., 2019). In peach, virus-induced gene silencing of Sepallata (PrupeSEP1) resulted in delayed softening of fruits (Li et al., 2017), and in cherry, silencing of PaMADS7 resulted in inhibited fruit ripening (Qi et al., 2020).
4.2.8 Resistance to common postharvest diseases
Strawberries suffer from numerous postharvest challenges. The thin epidermis of the strawberry receptacle leads to a propensity for mechanical damage, which can occur at all stages of growing, harvesting, and shipping (Gol et al., 2013; Sasaki et al., 2022; Quarshi et al., 2023). Strawberries also frequently undergo rapid softening after being harvested. The combination of these factors further translates into significant susceptibility to pathogens, including Botrytis cinerea, Rhizopus stolonifera, Mucor spp., Colletotrichum spp., and Penicillium spp. (Vu et al., 2011; Gol et al., 2013; Feliziani and Romanazzi, 2016). Of the numerous diseases known to affect strawberries after harvesting, Botrytis cinerea, also known as Botrytis fruit rot (BFR) or Gray Mold, is the primary disease responsible for postharvest loss. Botrytis cinerea was once considered the second most important plant fungal pathogen in the world due to its wide host range and ability to cause significant crop damage during both pre- and postharvest (Dean et al., 2012). Because postharvest diseases are major contributors to postharvest loss of strawberry, it is essential to continue improving postharvest disease resistance.
Overexpression of BRI1-Associated Kinase 1 (FaBAK1) and FaWRKY11 resulted in increased BFR resistance through promotion of defense pathways (Wang et al., 2021b; Li et al., 2023a), and RNAi of FaWRKY29, FaWRKY64, and FaWRKY25 resulted in significant increases in resistance to Botrytis cinerea through regulation of other defense-response genes (Wu et al., 2005a; Lee et al., 2023). A total of 247 WRKY TFs have been identified in Fragaria ×ananassa (Garrido-Gala et al., 2022). Members of the WRKY TF family have been characterized for various roles in biotic and abiotic stress response in several crop species (Wu et al., 2005a; Bai et al., 2018; Lee et al., 2023). Thus, further investigation of the roles of WRKY TFs in postharvest disease resistance, especially resistance to Botrytis cinerea, may be beneficial for the improvement of strawberry postharvest disease resistance. Knockout of PG1 increased resistance to BFR, possibly due to higher cell wall integrity and reduction of water loss associated with increased firmness (López-Casado et al., 2023). Similarly, RNAi of β-Glucosidase 3 (FaBG3) and Two-Pore K+ (FaTPK1) resulted in increased fruit firmness, delayed ripening, and increased resistance to BFR (Li et al., 2013; Wang et al., 2018), further supporting the impact of fruit firmness on BFR resistance. Multiple volatile compounds have also been tested for their effects on postharvest disease resistance. Methyl anthranilate and γ-decalactone, two major components of strawberry flavor, displayed antipathogenic activity against numerous common strawberry pathogens, including several of postharvest significance (Chambers et al., 2013). Additional exploration of the mechanisms underlying these increases in postharvest disease resistance may reveal targets for genome editing which will enable the improvement of resistance in parallel with other key fruit traits, such as firmness and flavor.
In tomato, knockout of Mitogen-Activated Protein Kinase (SlMAPK3), SlMYC2, and Autophagy-Related Gene 5 (SlATG5) resulted in increased susceptibility to BFR (Zhang et al., 2018; Shu et al., 2020; Li et al., 2023c), whereas knockout of tomato Phospholipase C2 (SlPLC2) increased resistance to BFR (Perk et al., 2023). Virus-induced gene silencing of RcWAK8 also significantly increased susceptibility to Botrytis cinerea in rose (Wang et al., 2023c). Transcriptomic analysis revealed that multiple FvWAK/WAKL genes were upregulated during Botrytis cinerea infection in strawberry, and which may contribute to BFR resistance (Wang et al., 2023c).
4.3 Potential applications of tools developed in strawberry to other rosaceous fruit crops
Rosaceae is composed of more than 100 genera and 3,000 species divided into several subfamilies (Potter et al., 2002; 2007; Shulaev et al., 2008), and wide genotypic and physiological variation within Rosaceae indicate a need for species-specific transformation protocols for crop improvement (Aldwinckle and Malnoy, 2009). However, if the focus is gene validation, then it would only be necessary to utilize a handful of species as model systems. Among the Rosaceae family, the genera Malus and Fragaria demonstrated the highest transformation efficiencies (Aldwinckle and Malnoy, 2009), indicating their potential value as model systems for reverse genetics in Rosaceae. Compared to Malus, Fragaria has a shorter transformation and regeneration timeline, with production of transgenic lines occurring in as little as 2 months, in addition to a shorter juvenile period (Folta et al., 2006; Aldwinckle and Malnoy, 2009). Fragaria vesca has several additional advantages over other plant model systems due to the ability to study mechanisms underlying fleshy fruit development, non-climacteric ripening, and unique metabolites, in addition to having one of the smallest genomes of cultivated plants (Folta and Davis, 2006; Shulaev et al., 2008). For these reasons, tools developed in Fragaria vesca may be beneficial to translational studies within Rosaceous species with long periods of juvenility, difficult transformation processes, or other barriers to genomics-assisted improvement. While certain disease resistance and woody plant architecture traits may be difficult to study in a Fragaria model (Aldwinckle and Malnoy, 2009), Vilanova et al. (2008) identified sufficient rates of genome synteny between Fragaria and Prunus to potentially allow for translational studies using marker genes and QTLs developed in strawberry. Others have found high rates of synteny between Rosa chiloensis and Fragaria vesca (Saint-Oyant et al., 2018), which further supports the use of strawberry as a model system for the development of various genomics and genomics-assisted tools for Rosaceae.
With recent increases in reference genome availability for various Rosaceous species came more interest in utilizing family-level approaches to identify loci underlying traits of agronomic interest. One such example of this application was reported by Zurn et al. (2020), in which sweetness-associated genes from Fragaria and Malus were used to identify a QTL associated with sugar content in blackberry, despite the established lack of blackberry-specific genomic resources. This report highlights the potential applications of marker genes and QTLs developed in widely studied species, such as strawberry, for genomics-assisted improvement of other Rosaceous species with fewer available genomic resources.
5 Regulation and commercialization of genome edited strawberry
As with other crops, commercial release of genome edited strawberry will depend on the regulatory frameworks established by individual countries or regions. Starting with the release of the framework 7 CFR Part3402 in 1987, genome edited and other genetically plants in the United States were regulated as part of a coordinated framework which includes the U.S Department of Agriculture Animal and Plant Health Inspection Service (USDA-APHIS), the U.S Environmental Protection Agency (EPA), and the U.S Food and Drug Administration (FDA) (United States Department of Agriculture, 2022; United States Food and Drug Administration, 2024). Seven CFR Part 340 underwent revisions in 2020, which included several updates to the existing regulatory system based on 3 decades of research and experience (United States Department of Agriculture, 2022). These updates provide a better breakdown of eligibility for non-regulated status. Application of CRISPR/Cas genome editing strategies can meet multiple of these eligibilities by introducing targeted single base-pair substitutions (7 CFR 340.1(b) (2)) or modifications which are present within the plant’s gene pool (7 CFR 340.1(b) (3)). In addition to the United States, several countries around the world have begun loosening restrictions on genome edited plants (Menz et al., 2020). While the European Union was slower to adopt looser regulations, the European Parliament recently voted to lessen regulatory oversight (Stokstad, 2024), representing a major step forward for global regulation of plant biotechnology.
At this time, there are no commercially available genome edited strawberry varieties. However, other edited species have undergone commercialization. In 2016, non-browning mushrooms were the first CRISPR/Cas9 genome edited organism to pass USDA regulation (Waltz, 2016). Pairwise recently released genome edited Brassica juncea with reduced pungency for improved flavor (Brown, 2023). Pairwise has also recently received nine new exemptions from USDA regulation for berry crops, bringing the company’s confirmed exemptions to 19 for berries and 21 in total (Barefoot, 2024). However, these genome edited berries have not been commercially released at the time of this review. These examples and others suggest a bright future for the de-regulation and commercialization of genome edited crops.
6 Conclusion and future perspectives
Cultivated strawberries are polyploid, highly heterozygous, and clonally propagated, which makes them difficult to improve through conventional methods. While application of genome editing in diploid woodland strawberry, Fragaria vesca, is helpful for validating gene function and identifying new candidate genes underlying traits of interest, the allo-octoploid cultivated strawberry, Fragaria ×ananassa, is the species of economic interest. Due to greater genomic complexity and the presence of homoeologous diploid subgenomes which are not closely related to Fragaria vesca, gene functions observed in woodland strawberry may not be completely conserved in octoploid strawberry. Thus, validation of gene function in the octoploid background is necessary for further elucidation of pathways underlying traits of interest. In turn, greater understanding of these pathways may then enable precise improvement of strawberry fruit quality and production traits through the application of genomics-assisted breeding techniques or the application of genome editing. Additionally, application of techniques such as base editing and prime editing to make precision nucleotide substitutions, or leveraging HDR to substitute alleles for superior variations, will enable additional opportunities for genomic improvement of strawberry.
While the application of genome editing for crop improvement is still relatively new to Fragaria ×ananassa and faces its own significant challenges, several examples of successful genome editing have been reported. These successful reports demonstrate the significant potential of genome editing in strawberry, highlighting the necessity to continue optimizing genome editing methods for improvement of economically important fruit traits. Based on the results of genome editing in Fragaria vesca, it may be beneficial to perform editing of orthologs of these genes in octoploid strawberry, to further confirm their roles in the commercially relevant species. Based on the results of transient analyses, some genes associated with desirable fruit traits may also be promising targets for genome editing. In the case of genes which have demonstrated negative effects on desirable traits when knocked out or transiently suppressed, further elucidation of their related pathways may reveal other associated genes which are better targets for application of genome editing and/or may permit fine-tuning of important fruit traits.
Author contributions
KV: Data curation, Formal Analysis, Writing–original draft, Writing–review and editing. FA: Writing–review and editing. TL: Writing–review and editing. SL: Conceptualization, Resources, Supervision, Writing–review and editing.
Funding
The author(s) declare that financial support was received for the research, authorship, and/or publication of this article. This research is supported by grants from the United Stated Department of Agriculture National Institute of Food and Agriculture (NIFA) Specialty Crops Research Initiative (#2022–51181-38328).
Acknowledgments
The authors thank the University of Florida (UF) Strawberry Breeding Program and Dr. Vance Whitaker for their technical and collaborative support for the strawberry research and this manuscript work. Additionally, thanks are extended to Dr. Antt Htet Wai from Strawberry Molecular Genetics and Genomics Program at UF for providing revisions and comments.
Conflict of interest
The authors declare that the research was conducted in the absence of any commercial or financial relationships that could be construed as a potential conflict of interest.
The author(s) declared that they were an editorial board member of Frontiers, at the time of submission. This had no impact on the peer review process and the final decision.
Publisher’s note
All claims expressed in this article are solely those of the authors and do not necessarily represent those of their affiliated organizations, or those of the publisher, the editors and the reviewers. Any product that may be evaluated in this article, or claim that may be made by its manufacturer, is not guaranteed or endorsed by the publisher.
Footnotes
1https://www.rosaceae.org/organism/Fragaria/x-ananassa?pane=resource-4
2https://www.ecfr.gov/current/title-7/subtitle-B/chapter-III/part-340
References
Afrin, S., Gasparrini, M., Forbes-Hernandez, T. Y., Reboredo-Rodriguez, P., Mezzetti, B., Varela-López, A., et al. (2016). Promising health benefits of the strawberry: a focus on clinical studies. J. Agric. Food Chem. 64 (22), 4435–4449. doi:10.1021/acs.jafc.6b00857
Agius, F., Amaya, I., Botella, M. A., and Valpuesta, V. (2005). Functional analysis of homologous and heterologous promoters in strawberry fruits using transient expression. J. Exp. Bot. 56 (409), 37–46. doi:10.1093/jxb/eri004
Agius, F., González-Lamothe, R., Caballero, J. L., Muñoz-Blanco, J., Botella, M. A., and Valpuesta, V. (2003). Engineering increased vitamin C levels in plants by overexpression of a D-galacturonic acid reductase. Nat. Biotechnol. 21 (2), 177–181. doi:10.1038/nbt777
Aguilar-Martínez, J. A., Poza-Carrión, C., and Cubas, P. (2007). Arabidopsis BRANCHED1 acts as an integrator of branching signals within axillary buds. Plant Cell 19 (2), 458–472. doi:10.1105/tpc.106.048934
Alarfaj, R., El-Soda, M., Antanaviciute, L., Vickerstaff, R., Hand, P., Harrison, R. J., et al. (2021). Mapping QTL underlying fruit quality traits in an F1 strawberry population. J. Hortic. Sci. Biotechnol. 96 (5), 634–645. doi:10.1080/14620316.2021.1912647
Aldwinckle, H., and Malnoy, M. (2009). Plant regeneration and transformation in the Rosaceae. Transgenic Plant J. 3 (Special Issue 1), 1–39.
Alger, E. I., Platts, A. E., Deb, S. K., Luo, X., Ou, S., Cao, Y., et al. (2021). Chromosome-scale genome for a red-fruited, perpetual flowering and runnerless woodland strawberry (fragaria vesca). Front. Genet. 12, 671371. doi:10.3389/fgene.2021.671371
Aliu, E., Azanu, M. K., Wang, K., and Lee, K. (2020). Generation of thymidine auxotrophic Agrobacterium tumefaciens strains for plant transformation. BioRxiv. doi:10.1101/2020.08.21.261941
Aliu, E., Lee, K., and Wang, K. (2022). CRISPR RNA-guided integrase enables high-efficiency targeted genome engineering in Agrobacterium tumefaciens. Plant Biotechnol. J. 20 (10), 1916–1927. doi:10.1111/pbi.13872
Anand, A., Bass, S. H., Wu, E., Wang, N., McBride, K. E., Annaluru, N., et al. (2018). An improved ternary vector system for Agrobacterium-mediated rapid maize transformation. Plant Mol. Biol. 97 (1), 187–200. doi:10.1007/s11103-018-0732-y
Anders, C., Bargsten, K., and Jinek, M. (2016). Structural plasticity of PAM recognition by engineered variants of the RNA-guided endonuclease Cas9. Mol. Cell 61 (6), 895–902. doi:10.1016/j.molcel.2016.02.020
Antunes, A. C. N., Acunha, T., dos, S., Perin, E. C., Rombaldi, C. V., Galli, V., et al. (2019). Untargeted metabolomics of strawberry (Fragaria x ananassa ‘Camarosa’) fruit from plants grown under osmotic stress conditions. J. Sci. Food Agric. 99 (15), 6973–6980. doi:10.1002/jsfa.9986
Anzalone, A. V., Koblan, L. W., and Liu, D. R. (2020). Genome editing with CRISPR-Cas nucleases, base editors, transposases and prime editors. Nat. Biotechnol. 38 (Issue 7), 824–844. doi:10.1038/s41587-020-0561-9
Azam, M., Ejaz, S., Naveed Ur Rehman, R., Khan, M., and Qadri, R. (2019). “Postharvest quality management of strawberries,” in Strawberry - pre- and post-harvest management techniques for higher fruit quality (London, United Kingdom: IntechOpen). doi:10.5772/intechopen.82341
Azameti, M. K., and Dauda, W. P. (2021). Base editing in plants: applications, challenges, and future prospects. Front. Plant Sci. 12, 664997. doi:10.3389/fpls.2021.664997
Bachelier, C., Graham, J., Machray, G., Du Manoir, J., Roucou, J. F., McNicol, R. J., et al. (1997). Integration of an invertase gene to control sucrose metabolism in strawberry cultivars. Acta Hortic. 439, 161–164. doi:10.17660/ActaHortic.1997.439.20
Bai, Y., Sunarti, S., Kissoudis, C., Visser, R. G. F., and van der Linden, C. G. (2018). The role of tomato WRKY genes in plant responses to combined abiotic and biotic stresses. Front. Plant Sci. 9, 801. doi:10.3389/fpls.2018.00801
Baldi, P., Orsucci, S., Moser, M., Brilli, M., Giongo, L., and Si-Ammour, A. (2018). Gene expression and metabolite accumulation during strawberry (Fragaria × ananassa) fruit development and ripening. Planta 248 (5), 1143–1157. doi:10.1007/s00425-018-2962-2
Barbey, C., Hogshead, M., Schwartz, A. E., Mourad, N., Verma, S., Lee, S., et al. (2020). The genetics of differential gene expression related to fruit traits in strawberry (fragaria ×ananassa). Front. Genet. 10, 1317. doi:10.3389/fgene.2019.01317
Barbey, C. R., Hogshead, M. H., Harrison, B., Schwartz, A. E., Verma, S., Oh, Y., et al. (2021). Genetic analysis of methyl anthranilate, mesifurane, linalool, and other flavor compounds in cultivated strawberry (fragaria × ananassa). Front. Plant Sci. 12, 615749. doi:10.3389/fpls.2021.615749
Barbey, C. R., Lee, S., Verma, S., Bird, K. A., Yocca, A. E., Edger, P. P., et al. (2019). Disease resistance genetics and genomics in octoploid strawberry. G3 Genes, Genomes, Genet. 9 (10), 3315–3332. doi:10.1534/g3.119.400597
Barceló, M., El-Mansouri, I., Mercado, J. A., Quesada, M. A., and Pliego Alfaro, F. (1998). Regeneration and transformation via Agrobacterium tumefaciens of the strawberry cultivar Chandler. Plant Cell, Tissue Organ Cult. 54 (1), 29–36. doi:10.1023/A:1006031527413
Barceló, M., Wallin, A., Medina, J. J., Gil-Ariza, D. J., López-Casado, G., Juarez, J., et al. (2019). Isolation and culture of strawberry protoplasts and field evaluation of regenerated plants. Sci. Hortic. 256, 108552. doi:10.1016/j.scienta.2019.108552
Barefoot, H. (2024). Pairwise earns USDA exemption confirmations for genomic edits in berries. Pairwise. Available at: https://www.pairwise.com/news/pairwise-earns-usda-exemption-confirmations-for-genomic-edits-in-berries.
Bassil, N. V., Davis, T. M., Zhang, H., Ficklin, S., Mittmann, M., Webster, T., et al. (2015). Development and preliminary evaluation of a 90 K Axiom® SNP array for the allo-octoploid cultivated strawberry Fragaria × ananassa. BMC Genomics 16 (1), 155. doi:10.1186/s12864-015-1310-1
Bernardo, R. (1994). Prediction of maize single-cross performance using RFLPs and information from related hybrids. Crop Sci. 34 (1), 20–25. doi:10.2135/cropsci1994.0011183X003400010003x
Bjorå, O. S., Brurberg, M. B., and Thorstensen, T. (2021). Functional analyses of Fvmyb46-CRISPR/Cas9-edited Fragaria vesca plants. Ås, Norway: Norwegian University of Life Sciences.
Bohra, A. (2013). Emerging paradigms in genomics-based crop improvement. Sci. World J. 2013, 585467. doi:10.1155/2013/585467
Bohra, A., Chand Jha, U., Godwin, I. D., and Kumar Varshney, R. (2020). Genomic interventions for sustainable agriculture. Plant Biotechnol. J. 18 (12), 2388–2405. doi:10.1111/pbi.13472
Brown, B. (2023). Pairwise introduces ConsciousTM greens into U.S. Restaurants. Pairwise. Available at: https://www.pairwise.com/news/pairwise-introduces-conscious-greens-into-u.s.-restaurants.
Capocasa, F., Scalzo, J., Mezzetti, B., and Battino, M. (2008). Combining quality and antioxidant attributes in the strawberry: the role of genotype. Food Chem. 111 (4), 872–878. doi:10.1016/j.foodchem.2008.04.068
Caruana, J. C., Sittmann, J. W., Wang, W., and Liu, Z. (2018). Suppressor of runnerless encodes a DELLA protein that controls runner formation for asexual reproduction in strawberry. Mol. Plant 11 (1), 230–233. doi:10.1016/j.molp.2017.11.001
Carvalho, R. F., Carvalho, S. D., O’Grady, K., and Folta, K. M. (2016). Agroinfiltration of strawberry fruit — a powerful transient expression system for gene validation. Curr. Plant Biol. 6, 19–37. doi:10.1016/j.cpb.2016.09.002
Castillejo, C., Waurich, V., Wagner, H., Ramos, R., Oiza, N., Muñoz, P., et al. (2020). Allelic variation of MYB10 Is the major force controlling natural variation in skin and flesh color in strawberry (Fragaria spp.) fruit. Plant Cell 32 (12), 3723–3749. doi:10.1105/tpc.20.00474
Castro, P., Bushakra, J. M., Stewart, P., Weebadde, C. K., Wang, D., Hancock, J. F., et al. (2015). Genetic mapping of day-neutrality in cultivated strawberry. Mol. Breed. 35 (2), 79. doi:10.1007/s11032-015-0250-4
Čermák, T., Baltes, N. J., Čegan, R., Zhang, Y., and Voytas, D. F. (2015). High-frequency, precise modification of the tomato genome. Genome Biol. 16 (1), 232. doi:10.1186/s13059-015-0796-9
Chai, Y., Jia, H., Li, C., Dong, Q., and Shen, Y. (2011). FaPYR1 is involved in strawberry fruit ripening. J. Exp. Bot. 62 (14), 5079–5089. doi:10.1093/jxb/err207
Chambers, A., Whitaker, V. M., Gibbs, B., Plotto, A., and Folta, K. M. (2012). Detection of the linalool-producing NES1 variant across diverse strawberry (Fragaria spp.) accessions. Plant Breed. 131 (3), 437–443. doi:10.1111/j.1439-0523.2012.01959.x
Chambers, A. H., Evans, S. A., and Folta, K. M. (2013). Methyl anthranilate and γ-decalactone inhibit strawberry pathogen growth and achene germination. J. Agric. Food Chem. 61 (51), 12625–12633. doi:10.1021/jf404255a
Chambers, A. H., Pillet, J., Plotto, A., Bai, J., Whitaker, V. M., and Folta, K. M. (2014). Identification of a strawberry flavor gene candidate using an integrated genetic-genomic-analytical chemistry approach. BMC Genomics 15 (1), 217. doi:10.1186/1471-2164-15-217
Chandler, C. K., Folta, K., Dale, A., Whitaker, V. M., and Herrington, M. (2012). “Strawberry,” in Fruit breeding. Editors M. L. Badenes, and D. H. Byrne (Springer US), 305–325. doi:10.1007/978-1-4419-0763-9_9
Chandra, S., Oh, Y., Han, H., Salinas, N., Anciro, A., Whitaker, V. M., et al. (2021). Comparative transcriptome analysis to identify candidate genes for FaRCg1 conferring resistance against Colletotrichum gloeosporioides in cultivated strawberry (fragaria × ananassa). Front. Genet. 12, 730444. doi:10.3389/fgene.2021.730444
Charrier, A., Vergne, E., Dousset, N., Richer, A., Petiteau, A., and Chevreau, E. (2019). Efficient targeted mutagenesis in apple and first time edition of pear using the CRISPR-Cas9 system. Front. Plant Sci. 10, 40. doi:10.3389/fpls.2019.00040
Chen, C., Zhang, X., Zhang, H., Ban, Z., Li, L., Dong, C., et al. (2019). Label-free quantitative proteomics to investigate the response of strawberry fruit after controlled ozone treatment. RSC Adv. 9 (2), 676–689. doi:10.1039/C8RA08405J
Chen, J., Li, S., He, Y., Li, J., and Xia, L. (2022). An update on precision genome editing by homology-directed repair in plants. Plant Physiol. 188 (4), 1780–1794. doi:10.1093/plphys/kiac037
Chen, X., Cai, W., Xia, J., Yu, H., Wang, Q., Pang, F., et al. (2020). Metabolomic and transcriptomic analyses reveal that blue light promotes chlorogenic acid synthesis in strawberry. J. Agric. Food Chem. 68 (44), 12485–12492. doi:10.1021/acs.jafc.0c05020
Chen, Y., Liu, L., Feng, Q., Liu, C., Bao, Y., Zhang, N., et al. (2023). FvWRKY50 is an important gene that regulates both vegetative growth and reproductive growth in strawberry. Hortic. Res. 10, uhad115. doi:10.1093/hr/uhad115
Cockerton, H. M., Vickerstaff, R. J., Karlström, A., Wilson, F., Sobczyk, M., He, J. Q., et al. (2018). Identification of powdery mildew resistance QTL in strawberry (Fragaria × ananassa). Theor. Appl. Genet. 131 (9), 1995–2007. doi:10.1007/s00122-018-3128-0
Collard, B. C. Y., and Mackill, D. J. (2008). Marker-assisted selection: an approach for precision plant breeding in the twenty-first century. Philosophical Trans. R. Soc. B Biol. Sci. 363 (1491), 557–572. doi:10.1098/rstb.2007.2170
Cong, L., Ran, F. A., Cox, D., Lin, S., Barretto, R., Habib, N., et al. (2013). Multiplex genome engineering using CRISPR/cas systems. Science 339 (6121), 819–823. doi:10.1126/science.1231143
Cordero de Mesa, M., Jiménez-Bermúdez, S., Pliego-Alfaro, F., Quesada, M. A., and Mercado, J. A. (2000). Agrobacterium cells as microprojectile coating: a novel approach to enhance stable transformation rates in strawberry. Funct. Plant Biol. 27 (12), 1093–1100. doi:10.1071/PP00025
Cui, L., Zheng, F., Wang, J., Zhang, C., Xiao, F., Ye, J., et al. (2020). miR156a-targeted SBP-Box transcription factor SlSPL13 regulates inflorescence morphogenesis by directly activating SFT in tomato. Plant Biotechnol. J. 18 (8), 1670–1682. doi:10.1111/pbi.13331
Dai, C., Yang, H., Tang, T., Ma, C., and Kang, C. (2020). “An effective CRISPR-cas9 technology for efficiently isolating transgene-free mutants in Arabidopsis, Brassica napus, strawberry, and soybean,” in CRISPR-cas methods. Editors M. T. Islam, P. K. Bhowmik, and K. A. Molla (Springer US), 99–115. doi:10.1007/978-1-0716-0616-2_7
Darwish, O., Shahan, R., Liu, Z., Slovin, J. P., and Alkharouf, N. W. (2015). Re-annotation of the woodland strawberry (Fragaria vesca) genome. BMC Genomics 16 (1), 29. doi:10.1186/s12864-015-1221-1
Dean, R., Van Kan, J. A. L., Pretorius, Z. A., Hammond-Kosack, K. E., Di Pietro, A., Spanu, P. D., et al. (2012). The Top 10 fungal pathogens in molecular plant pathology. Mol. Plant Pathol. 13 (4), 414–430. doi:10.1111/j.1364-3703.2011.00783.x
de Camacaro, M. E. P., Camacaro, G. J., Hadley, P., Battey, N. H., and Carew, J. G. (2002). Pattern of growth and development of the strawberry cultivars elsanta, bolero, and everest. J. Am. Soc. Hortic. Sci. Jashs 127 (6), 901–907. doi:10.21273/JASHS.127.6.901
de la Fuente, J. I., Amaya, I., Castillejo, C., Sánchez-Sevilla, J. F., Quesada, M. A., Botella, M. A., et al. (2006). The strawberry gene FaGAST affects plant growth through inhibition of cell elongation. J. Exp. Bot. 57 (10), 2401–2411. doi:10.1093/jxb/erj213
Deng, L., Wang, H., Sun, C., Li, Q., Jiang, H., Du, M., et al. (2018). Efficient generation of pink-fruited tomatoes using CRISPR/Cas9 system. J. Genet. Genomics 45 (1), 51–54. doi:10.1016/j.jgg.2017.10.002
Denoyes, B., Prohaska, A., Petit, J., and Rothan, C. (2023). Deciphering the genetic architecture of fruit color in strawberry. J. Exp. Bot. 74, 6306–6320. doi:10.1093/jxb/erad245
Devoghalaere, F., Doucen, T., Guitton, B., Keeling, J., Payne, W., Ling, T. J., et al. (2012). A genomics approach to understanding the role of auxin in apple (Malus x domestica)fruit size control. BMC Plant Biol. 12 (1), 7. doi:10.1186/1471-2229-12-7
Diamanti, J., Capocasa, F., Balducci, F., Battino, M., Hancock, J., and Mezzetti, B. (2012). Increasing strawberry fruit sensorial and nutritional quality using wild and cultivated germplasm. PLOS ONE 7 (10), e46470. doi:10.1371/journal.pone.0046470
Diamanti, J., Mazzoni, L., Balducci, F., Cappelletti, R., Capocasa, F., Battino, M., et al. (2014). Use of wild genotypes in breeding program increases strawberry fruit sensorial and nutritional quality. J. Agric. Food Chem. 62 (18), 3944–3953. doi:10.1021/jf500708x
Doudna, J. A., and Charpentier, E. (2014). Genome editing. The new frontier of genome engineering with CRISPR-Cas9. Science 346 (6213), 1258096. doi:10.1126/science.1258096
Duan, K., Zhao, Y. J., Li, Z. Y., Zou, X. H., Yang, J., Guo, C. L., et al. (2021). A strategy for the production and molecular validation of agrobacterium-mediated intragenic octoploid strawberry. Plants 10 (11), 2229. doi:10.3390/plants10112229
Edger, P. P., McKain, M. R., Yocca, A. E., Knapp, S. J., Qiao, Q., and Zhang, T. (2020). Reply to: revisiting the origin of octoploid strawberry. Nat. Genet. 52 (1), 5–7. doi:10.1038/s41588-019-0544-2
Edger, P. P., Poorten, T. J., VanBuren, R., Hardigan, M. A., Colle, M., McKain, M. R., et al. (2019). Origin and evolution of the octoploid strawberry genome. Nat. Genet. 51 (3), 541–547. doi:10.1038/s41588-019-0356-4
Edger, P. P., VanBuren, R., Colle, M., Poorten, T. J., Wai, C. M., Niederhuth, C. E., et al. (2018). Single-molecule sequencing and optical mapping yields an improved genome of woodland strawberry (Fragaria vesca) with chromosome-scale contiguity. GigaScience 7 (2), 1–7. doi:10.1093/gigascience/gix124
El-Mounadi, K., Morales-Floriano, M. L., and Garcia-Ruiz, H. (2020). Principles, applications, and biosafety of plant genome editing using CRISPR-cas9. Front. Plant Sci. 11, 56. doi:10.3389/fpls.2020.00056
Fan, Z., Tieman, D. M., Knapp, S. J., Zerbe, P., Famula, R., Barbey, C. R., et al. (2022). A multi-omics framework reveals strawberry flavor genes and their regulatory elements. New Phytol. 236 (3), 1089–1107. doi:10.1111/nph.18416
Fan, Z., Verma, S., Lee, H., Jang, Y. J., Wang, Y., Lee, S., et al. (2023). Strawberry soluble solids QTL with inverse effects on yield. Hortic. Res. 11, uhad271. doi:10.1093/hr/uhad271
FAO (2023). FAOSTAT. License: CC BY-NC-SA 3.0 IGO. Available at: https://www.fao.org/faostat/en/#data/QV (Accessed September 26, 2023).
Feliziani, E., and Romanazzi, G. (2016). Postharvest decay of strawberry fruit: etiology, epidemiology, and disease management. J. Berry Res. 6 (1), 47–63. doi:10.3233/JBR-150113
Feng, C., Wang, J., Harris, A. J., Folta, K. M., Zhao, M., and Kang, M. (2021a). Tracing the diploid ancestry of the cultivated octoploid strawberry. Mol. Biol. Evol. 38 (2), 478–485. doi:10.1093/molbev/msaa238
Feng, J., Cheng, L., Zhu, Z., Yu, F., Dai, C., Liu, Z., et al. (2021b). GRAS transcription factor LOSS of AXILLARY MERISTEMS is essential for stamen and runner formation in wild strawberry. Plant Physiol. 186 (4), 1970–1984. doi:10.1093/plphys/kiab184
Feng, J., Dai, C., Luo, H., Han, Y., Liu, Z., and Kang, C. (2019). Reporter gene expression reveals precise auxin synthesis sites during fruit and root development in wild strawberry. J. Exp. Bot. 70 (2), 563–574. doi:10.1093/jxb/ery384
Filler Hayut, S., Melamed Bessudo, C., and Levy, A. A. (2017). Targeted recombination between homologous chromosomes for precise breeding in tomato. Nat. Commun. 8 (1), 15605. doi:10.1038/ncomms15605
Finlayson, S. A. (2007). Arabidopsis TEOSINTE BRANCHED1-LIKE 1 regulates axillary bud outgrowth and is homologous to monocot TEOSINTE BRANCHED1. Plant Cell Physiology 48 (5), 667–677. doi:10.1093/pcp/pcm044
Folta, K. M., and Davis, T. M. (2006). Strawberry genes and genomics. Crit. Rev. Plant Sci. 25 (5), 399–415. doi:10.1080/07352680600824831
Folta, K. M., Dhingra, A., Howard, L., Stewart, P. J., and Chandler, C. K. (2006). Characterization of LF9, an octoploid strawberry genotype selected for rapid regeneration and transformation. Planta 224 (5), 1058–1067. doi:10.1007/s00425-006-0278-0
Fuentes, L., Figueroa, C. R., and Valdenegro, M. (2019). Recent advances in hormonal regulation and cross-talk during non-climacteric fruit development and ripening. Horticulturae 5 (Issue 2), 45. doi:10.3390/horticulturae5020045
Gao, C. (2021). Genome engineering for crop improvement and future agriculture. Cell 184 (6), 1621–1635. doi:10.1016/j.cell.2021.01.005
Gao, Q., Luo, H., Li, Y., Liu, Z., and Kang, C. (2020). Genetic modulation of RAP alters fruit coloration in both wild and cultivated strawberry. Plant Biotechnol. J. 18 (7), 1550–1561. doi:10.1111/pbi.13317
García-Gago, J. A., Posé, S., Muñoz-Blanco, J., Quesada, M. A., and Mercado, J. A. (2009). The polygalacturonase FaPG1 gene plays a key role in strawberry fruit softening. Plant Signal. Behav. 4 (8), 766–768. doi:10.1104/pp.109.138297
Garrido-Gala, J., Higuera, J.-J., Rodríguez-Franco, A., Muñoz-Blanco, J., Amil-Ruiz, F., and Caballero, J. L. (2022). A comprehensive study of the WRKY transcription factor family in strawberry. Plants 11 (12), 1585. doi:10.3390/plants11121585
Gaston, A., Osorio, S., Denoyes, B., and Rothan, C. (2020). Applying the solanaceae strategies to strawberry crop improvement. Trends Plant Sci. 25 (2), 130–140. doi:10.1016/j.tplants.2019.10.003
Gaston, A., Potier, A., Alonso, M., Sabbadini, S., Delmas, F., Tenreira, T., et al. (2021). The FveFT2 florigen/FveTFL1 antiflorigen balance is critical for the control of seasonal flowering in strawberry while FveFT3 modulates axillary meristem fate and yield. New Phytol. 232 (1), 372–387. doi:10.1111/nph.17557
Gezan, S. A., Osorio, L. F., Verma, S., and Whitaker, V. M. (2017). An experimental validation of genomic selection in octoploid strawberry. Hortic. Res. 4, 16070. doi:10.1038/hortres.2016.70
Ghogare, R., Williamson-Benavides, B., Ramírez-Torres, F., and Dhingra, A. (2020). CRISPR-associated nucleases: the Dawn of a new age of efficient crop improvement. Transgenic Res. 29 (1), 1–35. doi:10.1007/s11248-019-00181-y
Giampieri, F., Alvarez-Suarez, J. M., and Battino, M. (2014). Strawberry and human health: effects beyond antioxidant activity. J. Agric. Food Chem. 62 (18), 3867–3876. doi:10.1021/jf405455n
Giampieri, F., Alvarez-Suarez, J. M., Mazzoni, L., Romandini, S., Bompadre, S., Diamanti, J., et al. (2013). The potential impact of strawberry on human health. Nat. Prod. Res. 27 (4–5), 448–455. doi:10.1080/14786419.2012.706294
Giampieri, F., Gasparrini, M., Forbes-Hernandez, T. Y., Mazzoni, L., Capocasa, F., Sabbadini, S., et al. (2018). Overexpression of the anthocyanidin synthase gene in strawberry enhances antioxidant capacity and cytotoxic effects on human hepatic cancer cells. J. Agric. Food Chem. 66 (3), 581–592. doi:10.1021/acs.jafc.7b04177
Giampieri, F., Tulipani, S., Alvarez-Suarez, J. M., Quiles, J. L., Mezzetti, B., and Battino, M. (2012). The strawberry: composition, nutritional quality, and impact on human health. Nutrition 28 (1), 9–19. doi:10.1016/j.nut.2011.08.009
Goddard, M. E., and Hayes, B. J. (2007). Genomic selection. J. Animal Breed. Genet. 124 (6), 323–330. doi:10.1111/j.1439-0388.2007.00702.x
Gol, N. B., Patel, P. R., and Rao, T. V. R. (2013). Improvement of quality and shelf-life of strawberries with edible coatings enriched with chitosan. Postharvest Biol. Technol. 85, 185–195. doi:10.1016/j.postharvbio.2013.06.008
Gou, Y.-J., Li, Y.-L., Bi, P.-P., Wang, D.-J., Ma, Y.-Y., Hu, Y., et al. (2020). Optimization of the protoplast transient expression system for gene functional studies in strawberry (Fragaria vesca). Plant Cell, Tissue Organ Cult. (PCTOC) 141 (1), 41–53. doi:10.1007/s11240-020-01765-x
Graham, J., McNicol, R. J., and Greig, K. (1995). Towards genetic based insect resistance in strawberry using the Cowpea trypsin inhibitor gene. Ann. Appl. Biol. 127 (1), 163–173. doi:10.1111/j.1744-7348.1995.tb06661.x
Greb, T., Clarenz, O., Schäfer, E., Müller, D., Herrero, R., Schmitz, G., et al. (2003). Molecular analysis of the LATERAL SUPPRESSOR gene in Arabidopsis reveals a conserved control mechanism for axillary meristem formation. Genes Dev. 17 (9), 1175–1187. doi:10.1101/gad.260703
Gruchała, A., Korbin, M., and Żurawicz, E. (2004a). Conditions of transformation and regeneration of `Induka’ and `Elista’ strawberry plants. Plant Cell, Tissue Organ Cult. 79 (2), 153–160. doi:10.1007/s11240-004-0655-y
Gruchała, A., Korbin, M., and Żurawicz, E. (2004b). Suitability of selected strawberry cultivars for genome modifications by agrobacterium tumefaciens. Acta Hortic. 663, 491–494. doi:10.17660/ActaHortic.2004.663.86
Gu, X., Liu, L., and Zhang, H. (2021). Transgene-free genome editing in plants. Front. Genome Ed. 3, 805317. doi:10.3389/fgeed.2021.805317
Guilinger, J. P., Thompson, D. B., and Liu, D. R. (2014). Fusion of catalytically inactive Cas9 to FokI nuclease improves the specificity of genome modification. Nat. Biotechnol. 32 (6), 577–582. doi:10.1038/nbt.2909
Haddadi, F., Aziz, M. A., Abdullah, S. N. A., Tan, S. G., and Kamaladini, H. (2015). An efficient Agrobacterium-mediated transformation of strawberry cv. Camarosa by a dual plasmid system. Molecules 20 (3), 3647–3666. doi:10.3390/molecules20033647
Han, H., Barbey, C. R., Fan, Z., Verma, S., Whitaker, V. M., and Lee, S. (2022). Telomere-to-Telomere and haplotype-phased genome assemblies of the heterozygous octoploid “Florida brilliance” strawberry (fragaria ×ananassa). biorxiv. doi:10.1101/2022.10.05.509768
Han, J., Li, X., Li, W., Yang, Q., Li, Z., Cheng, Z., et al. (2023). Isolation and preliminary functional analysis of FvICE1, involved in cold and drought tolerance in Fragaria vesca through overexpression and CRISPR/Cas9 technologies. Plant Physiology Biochem. 196, 270–280. doi:10.1016/j.plaphy.2023.01.048
Han, Y., Dang, R., Li, J., Jiang, J., Zhang, N., Jia, M., et al. (2015). Sucrose nonfermenting1-related protein kinase2.6, an ortholog of open stomata1, is a negative regulator of strawberry fruit development and ripening. Plant Physiol. 167 (3), 915–930. doi:10.1104/pp.114.251314
Hancock, J., Sjulin, T. M., and Lobos, G. (2008). “Strawberries,” in Temperate fruit crop breeding, 393–438. doi:10.1007/978-1-4020-6907-9-13
Hardigan, M. A., Feldmann, M. J., Pincot, D. D. A., Famula, R. A., Vachev, M. V., Madera, M. A., et al. (2021a). Blueprint for phasing and assembling the genomes of heterozygous polyploids: application to the octoploid genome of strawberry. bioRxiv. doi:10.1101/2021.11.03.467115
Hardigan, M. A., Lorant, A., Pincot, D. D. A., Feldmann, M. J., Famula, R. A., Acharya, C. B., et al. (2021b). Unraveling the complex hybrid ancestry and domestication history of cultivated strawberry. Mol. Biol. Evol. 38 (6), 2285–2305. doi:10.1093/molbev/msab024
Harrington, L. B., Burstein, D., Chen, J. S., Paez-Espino, D., Ma, E., Witte, I. P., et al. (2018). Programmed DNA destruction by miniature CRISPR-Cas14 enzymes. Science 362 (6416), 839–842. doi:10.1126/science.aav4294
Härtl, K., Kalinowski, G., Hoffmann, T., Preuss, A., and Schwab, W. (2017). RNAi-mediated endogene silencing in strawberry fruit: detection of primary and secondary siRNAs by deep sequencing. Plant Biotechnol. J. 15 (5), 658–668. doi:10.1111/pbi.12664
Hartmann, H. T. (1947). Some effects of temperature and photoperiod on flower formation and runner production in the strawberry. Plant Physiol. 22 (4), 407–420. doi:10.1104/pp.22.4.407
Havlicek, S., Shen, Y., Alpagu, Y., Bruntraeger, M. B., Zufir, N. B. M., Phuah, Z. Y., et al. (2017). Re-Engineered RNA-guided FokI-nucleases for improved genome editing in human cells. Mol. Ther. 25 (2), 342–355. doi:10.1016/j.ymthe.2016.11.007
He, Y., Zhu, M., Wang, L., Wu, J., Wang, Q., Wang, R., et al. (2018). Programmed self-elimination of the CRISPR/Cas9 construct greatly accelerates the isolation of edited and transgene-free rice plants. Mol. Plant 11 (9), 1210–1213. doi:10.1016/j.molp.2018.05.005
He, Y., Zhu, M., Wang, L., Wu, J., Wang, Q., Wang, R., et al. (2019). Improvements of TKC technology accelerate isolation of transgene-free CRISPR/Cas9-Edited rice plants. Rice Sci. 26 (2), 109–117. doi:10.1016/j.rsci.2018.11.001
Hirakawa, H., Shirasawa, K., Kosugi, S., Tashiro, K., Nakayama, S., Yamada, M., et al. (2014). Dissection of the octoploid strawberry genome by deep sequencing of the genomes of fragaria species. DNA Res. 21 (2), 169–181. doi:10.1093/dnares/dst049
Hoffmann, T., Kalinowski, G., and Schwab, W. (2006). RNAi-induced silencing of gene expression in strawberry fruit (Fragaria x ananassa) by agroinfiltration: a rapid assay for gene function analysis. Plant J. 48 (5), 818–826. doi:10.1111/j.1365-313X.2006.02913.x
Hoffmann, T., Kurtzer, R., Skowranek, K., Kießling, P., Fridman, E., Pichersky, E., et al. (2011). Metabolic engineering in strawberry fruit uncovers a dormant biosynthetic pathway. Metab. Eng. 13 (5), 527–531. doi:10.1016/j.ymben.2011.06.002
Hollender, C. A., Geretz, A. C., Slovin, J. P., and Liu, Z. (2012). Flower and early fruit development in a diploid strawberry, Fragaria vesca. Planta 235 (6), 1123–1139. doi:10.1007/s00425-011-1562-1
Hortyński, J. A., Żebrowska, J., Gawroński, J., and Hulewicz, T. (1991). Factors influencing fruit size in the strawberry (Fragaria ananassa Duch.). Euphytica 56 (1), 67–74. doi:10.1007/BF00041745
Hossain, M. R., Natarajan, S., Kim, H.-T., Jesse, D. M. I., Lee, C.-G., Park, J.-I., et al. (2019). High density linkage map construction and QTL mapping for runner production in allo-octoploid strawberry Fragaria × ananassa based on ddRAD-seq derived SNPs. Sci. Rep. 9 (1), 3275. doi:10.1038/s41598-019-39808-9
Hu, J., Li, X., and Sun, T. (2023). Four class A AUXIN RESPONSE FACTORs promote tomato fruit growth despite suppressing fruit set. Nat. Plants 9 (5), 706–719. doi:10.1038/s41477-023-01396-y
Huang, T.-K., and Puchta, H. (2021). Novel CRISPR/Cas applications in plants: from prime editing to chromosome engineering. Transgenic Res. 30 (4), 529–549. doi:10.1007/s11248-021-00238-x
Husaini, A. M. (2010). Pre- and post-agroinfection strategies for efficient leaf disk transformation and regeneration of transgenic strawberry plants. Plant Cell Rep. 29 (1), 97–110. doi:10.1007/s00299-009-0801-4
Hytönen, T., Elomaa, P., Moritz, T., and Junttila, O. (2009). Gibberellin mediates daylength-controlled differentiation of vegetative meristems in strawberry (Fragaria x ananassa Duch). BMC Plant Biol. 9, 18. doi:10.1186/1471-2229-9-18
Hytönen, T., and Kurokura, T. (2020). Control of flowering and runnering in strawberry. Hortic. J. 89 (2), 96–107. doi:10.2503/hortj.UTD-R011
Iwata, H., Gaston, A., Remay, A., Thouroude, T., Jeauffre, J., Kawamura, K., et al. (2012). The TFL1 homologue KSN is a regulator of continuous flowering in rose and strawberry. Plant J. 69 (1), 116–125. doi:10.1111/j.1365-313X.2011.04776.x
Jamal Uddin, A., Hossan, M., Islam, M., Ahsan, M., and Mehraj, H. (2012). Strawberry growth and yield responses to gibberellic acid concentrations. J. Expt. Biosci. 3 (2), 51–56. Available at: https://ssrn.com/abstract=3570408.
James, D. J., Passey, A. J., and Barbara, D. J. (1990). Agrobacterium-mediated transformation of the cultivated strawberry (Fragaria × anannassa duch.) using disarmed binary vectors. Plant Sci. 69 (1), 79–94. doi:10.1016/0168-9452(90)90106-X
Jeong, S. Y., Ahn, H., Ryu, J., Oh, Y., Sivanandhan, G., Won, K.-H., et al. (2019). Generation of early-flowering Chinese cabbage (Brassica rapa spp. pekinensis) through CRISPR/Cas9-mediated genome editing. Plant Biotechnol. Rep. 13 (5), 491–499. doi:10.1007/s11816-019-00566-9
Jiang, F., Wang, J.-Y., Jia, H.-F., Jia, W.-S., Wang, H.-Q., and Xiao, M. (2013). RNAi-mediated silencing of the flavanone 3-hydroxylase gene and its effect on flavonoid biosynthesis in strawberry fruit. J. Plant Growth Regul. 32 (1), 182–190. doi:10.1007/s00344-012-9289-1
Jiang, L., Yue, M., Liu, Y., Zhang, N., Lin, Y., Zhang, Y., et al. (2023). A novel R2R3-MYB transcription factor FaMYB5 positively regulates anthocyanin and proanthocyanidin biosynthesis in cultivated strawberries (Fragaria × ananassa). Plant Biotechnol. J. 21 (6), 1140–1158. doi:10.1111/pbi.14024
Jiménez-Bermúdez, S., Redondo-Nevado, J., Muñoz-Blanco, J., Caballero, J. L., López-Aranda, J. M., Valpuesta, V., et al. (2002). Manipulation of strawberry fruit softening by antisense expression of a pectate lyase gene. Plant Physiol. 128 (2), 751–759. doi:10.1104/pp.010671
Jin, X., Du, H., Zhu, C., Wan, H., Liu, F., Ruan, J., et al. (2023). Haplotype-resolved genomes of wild octoploid progenitors illuminate genomic diversifications from wild relatives to cultivated strawberry. Nat. Plants 9 (8), 1252–1266. doi:10.1038/s41477-023-01473-2
Jiu, S., Haider, M. S., Kurjogi, M. M., Zhang, K., Zhu, X., and Fang, J. (2018). Genome-wide characterization and expression analysis of sugar transporter family genes in woodland strawberry. Plant Genome 11 (3), 170103. doi:10.3835/plantgenome2017.11.0103
Joldersma, D., Sadowski, N., Timp, W., and Liu, Z. (2022). Assembly and annotation of Fragaria vesca “Yellow Wonder” genome, a model diploid strawberry for molecular genetic research. Fruit Res. 2 (1), 1–5. doi:10.48130/frures-2022-0013
Jung, H.-J., Veerappan, K., Natarajan, S., Jeong, N., Hwang, I., Nagano, S., et al. (2017). A system for distinguishing octoploid strawberry cultivars using high-throughput SNP genotyping. Trop. Plant Biol. 10 (2), 68–76. doi:10.1007/s12042-017-9185-8
Kadomura-Ishikawa, Y., Miyawaki, K., Takahashi, A., and Noji, S. (2015). RNAi-mediated silencing and overexpression of the FaMYB1 gene and its effect on anthocyanin accumulation in strawberry fruit. Biol. Plant. 59 (4), 677–685. doi:10.1007/s10535-015-0548-4
Kang, C., Darwish, O., Geretz, A., Shahan, R., Alkharouf, N., and Liu, Z. (2013). Genome-scale transcriptomic insights into early-stage fruit development in woodland strawberry fragaria vesca. Plant Cell 25 (6), 1960–1978. doi:10.1105/tpc.113.111732
Kantor, A., McClements, M. E., and Maclaren, R. E. (2020). Crispr-cas9 dna base-editing and prime-editing. Int. J. Mol. Sci. 21 (17), 6240–6322. doi:10.3390/ijms21176240
Kapoor, L., Simkin, A. J., George Priya Doss, C., and Siva, R. (2022). Fruit ripening: dynamics and integrated analysis of carotenoids and anthocyanins. BMC Plant Biol. 22 (1), 27. doi:10.1186/s12870-021-03411-w
Karlson, D., Mojica, J. P., Poorten, T. J., Lawit, S. J., Jali, S., Chauhan, R. D., et al. (2022). Targeted mutagenesis of the multicopy myrosinase gene family in allotetraploid Brassica juncea reduces pungency in fresh leaves across environments. Plants 11 (19), 2494. doi:10.3390/plants11192494
Komor, A. C., Badran, A. H., and Liu, D. R. (2017). CRISPR-based technologies for the manipulation of eukaryotic genomes. Cell 168 (Issues 1–2), 20–36. doi:10.1016/j.cell.2016.10.044
Komor, A. C., Kim, Y. B., Packer, M. S., Zuris, J. A., and Liu, D. R. (2016). Programmable editing of a target base in genomic DNA without double-stranded DNA cleavage. Nature 533 (7603), 420–424. doi:10.1038/nature17946
Koskela, E. A., Mouhu, K., Albani, M. C., Kurokura, T., Rantanen, M., Sargent, D. J., et al. (2012). Mutation in TERMINAL FLOWER1 reverses the photoperiodic requirement for flowering in the wild strawberry fragaria vesca. Plant Physiol. 159 (3), 1043–1054. doi:10.1104/pp.112.196659
Lee, H. E., Manivannan, A., Lee, S. Y., Han, K., Yeum, J. G., Jo, J., et al. (2021). Chromosome level assembly of homozygous inbred line ‘wongyo 3115’ facilitates the construction of a high-density linkage map and identification of QTLs associated with fruit firmness in octoploid strawberry (fragaria × ananassa). Front. Plant Sci. 12, 696229. doi:10.3389/fpls.2021.696229
Lee, J., Kim, H.-B., Noh, Y.-H., Min, S. R., Lee, H.-S., Jung, J., et al. (2018). Sugar content and expression of sugar metabolism-related gene in strawberry fruits from various cultivars. J. Plant Biotechnol. 45 (2), 90–101. doi:10.5010/JPB.2018.45.2.090
Lee, M. B., Han, H., and Lee, S. (2023). The role of WRKY transcription factors, FaWRKY29 and FaWRKY64, for regulating Botrytis fruit rot resistance in strawberry (Fragaria × ananassa Duch.). BMC Plant Biol. 23 (1), 420. doi:10.1186/s12870-023-04426-1
Li, C., Jia, H., Chai, Y., and Shen, Y. (2011). Abscisic acid perception and signaling transduction in strawberry: a model for non-climacteric fruit ripening. Plant Signal. Behav. 6 (12), 1950–1953. doi:10.4161/psb.6.12.18024
Li, C., Wang, K., Tan, M., Lei, C., and Cao, S. (2023a). Involvement of a receptor-like kinase complex of FvFLS2 and FvBAK1 in brassinosteroids-induced immunity in postharvest strawberry fruit. Postharvest Biol. Technol. 198, 112266. doi:10.1016/j.postharvbio.2023.112266
Li, C., Yamagishi, N., Kasajima, I., and Yoshikawa, N. (2019a). Virus-induced gene silencing and virus-induced flowering in strawberry (Fragaria × ananassa) using apple latent spherical virus vectors. Hortic. Res. 6, 18. doi:10.1038/s41438-018-0106-2
Li, J., Li, F., Qian, M., Han, M., Liu, H., Zhang, D., et al. (2017). Characteristics and regulatory pathway of the PrupeSEP1 SEPALLATA gene during ripening and softening in peach fruits. Plant Sci. 257, 63–73. doi:10.1016/j.plantsci.2017.01.004
Li, Q., Ji, K., Sun, Y., Luo, H., Wang, H., and Leng, P. (2013). The role of FaBG3 in fruit ripening and B. cinerea fungal infection of strawberry. Plant J. 76 (1), 24–35. doi:10.1111/tpj.12272
Li, W., Zhang, J., Sun, H., Wang, S., Chen, K., Liu, Y., et al. (2018a). FveRGA1, encoding a DELLA protein, negatively regulates runner production in Fragaria vesca. Planta 247 (4), 941–951. doi:10.1007/s00425-017-2839-9
Li, X., Martín-Pizarro, C., Zhou, L., Hou, B., Wang, Y., Shen, Y., et al. (2023b). Deciphering the regulatory network of the NAC transcription factor FvRIF, a key regulator of strawberry (Fragaria vesca) fruit ripening. Plant Cell 35, 4020–4045. doi:10.1093/plcell/koad210
Li, Y., Pi, M., Gao, Q., Liu, Z., and Kang, C. (2019b). Updated annotation of the wild strawberry Fragaria vesca V4 genome. Hortic. Res. 6 (1), 61. doi:10.1038/s41438-019-0142-6
Li, Y., Shu, P., Xiang, L., Sheng, J., and Shen, L. (2023c). CRISPR/Cas9-Mediated SlATG5 mutagenesis reduces the resistance of tomato fruit to Botrytis cinerea. Foods 12 (14), 2750. doi:10.3390/foods12142750
Li, Y., Wei, W., Feng, J., Luo, H., Pi, M., Liu, Z., et al. (2018b). Genome re-annotation of the wild strawberry Fragaria vesca using extensive Illumina-and SMRT-based RNA-seq datasets. DNA Res. 25 (1), 61–70. doi:10.1093/dnares/dsx038
Liao, X., Li, M., Liu, B., Yan, M., Yu, X., Zi, H., et al. (2018). Interlinked regulatory loops of ABA catabolism and biosynthesis coordinate fruit growth and ripening in woodland strawberry. Proc. Natl. Acad. Sci. U. S. A. 115 (49), E11542–E11550. doi:10.1073/pnas.1812575115
Lin, Q., Zong, Y., Xue, C., Wang, S., Jin, S., Zhu, Z., et al. (2020). Prime genome editing in rice and wheat. Nat. Biotechnol. 38 (5), 582–585. doi:10.1038/s41587-020-0455-x
Lin, X., Xiao, M., Luo, Y., Wang, J., and Wang, H. (2013). The effect of RNAi-induced silencing of FaDFR on anthocyanin metabolism in strawberry (Fragaria×ananassa) fruit. Sci. Hortic. 160, 123–128. doi:10.1016/j.scienta.2013.05.024
Liston, A., Wei, N., Tennessen, J. A., Li, J., Dong, M., and Ashman, T.-L. (2020). Revisiting the origin of octoploid strawberry. Nat. Genet. 52 (1), 2–4. doi:10.1038/s41588-019-0543-3
Liu, H., Xie, W.-F., Zhang, L., Valpuesta, V., Ye, Z.-W., Gao, Q.-H., et al. (2014). Auxin biosynthesis by the YUCCA6 flavin monooxygenase gene in woodland strawberry. J. Integr. Plant Biol. 56 (4), 350–363. doi:10.1111/jipb.12150
Liu, H., Ying, Y.-Y., Zhang, L., Gao, Q.-H., Li, J., Zhang, Z., et al. (2012). Isolation and characterization of two YUCCA flavin monooxygenase genes from cultivated strawberry (Fragaria × ananassa Duch.). Plant Cell Rep. 31 (8), 1425–1435. doi:10.1007/s00299-012-1258-4
Liu, J. J., Orlova, N., Oakes, B. L., Ma, E., Spinner, H. B., Baney, K. L. M., et al. (2019). CasX enzymes comprise a distinct family of RNA-guided genome editors. Nature 566 (7743), 218–223. doi:10.1038/s41586-019-0908-x
Liu, T., Li, M., Liu, Z., Ai, X., and Li, Y. (2021). Reannotation of the cultivated strawberry genome and establishment of a strawberry genome database. Hortic. Res. 8 (1), 41. doi:10.1038/s41438-021-00476-4
Liu, W., Rudis, M. R., Cheplick, M. H., Millwood, R. J., Yang, J.-P., Ondzighi-Assoume, C. A., et al. (2020). Lipofection-mediated genome editing using DNA-free delivery of the Cas9/gRNA ribonucleoprotein into plant cells. Plant Cell Rep. 39 (2), 245–257. doi:10.1007/s00299-019-02488-w
Liu, Z., Liang, T., and Kang, C. (2023). Molecular bases of strawberry fruit quality traits: advances, challenges, and opportunities. Plant Physiol. 193 (2), 900–914. doi:10.1093/plphys/kiad376
López-Casado, G., Sánchez-Raya, C., Ric-Varas, P. D., Paniagua, C., Blanco-Portales, R., Muñoz-Blanco, J., et al. (2023). CRISPR/Cas9 editing of the polygalacturonase FaPG1 gene improves strawberry fruit firmness. Hortic. Res. 10 (3), uhad011. doi:10.1093/hr/uhad011
Lunkenbein, S., Coiner, H., de Vos, C. H. R., Schaart, J. G., Boone, M. J., Krens, F. A., et al. (2006). Molecular characterization of a stable antisense chalcone synthase phenotype in strawberry (Fragaria x ananassa). J. Agric. Food Chem. 54 (6), 2145–2153. doi:10.1021/jf052574z
Luo, H., Dai, C., Li, Y., Feng, J., Liu, Z., and Kang, C. (2018). Reduced Anthocyanins in Petioles codes for a GST anthocyanin transporter that is essential for the foliage and fruit coloration in strawberry. J. Exp. Bot. 69 (10), 2595–2608. doi:10.1093/jxb/ery096
Lv, J., Zheng, T., Song, Z., Pervaiz, T., Dong, T., Zhang, Y., et al. (2022). Strawberry proteome responses to controlled hot and cold stress partly mimic post-harvest storage temperature effects on fruit quality. Front. Nutr. 8, 812666. doi:10.3389/fnut.2021.812666
Lyu, K., Xiao, J., Lyu, S., and Liu, R. (2023). Comparative analysis of transposable elements in strawberry genomes of different ploidy levels. Int. J. Mol. Sci. 24 (23), 16935. doi:10.3390/ijms242316935
Mackenzie, S. J., Chandler, C. K., Hasing, T., and Whitaker, V. M. (2011). The role of temperature in the late-season decline in soluble solids content of strawberry fruit in a subtropical production system. HORTSCIENCE 46 (Issue 11), 1562–1566. doi:10.21273/hortsci.46.11.1562
Mali, P., Yang, L., Esvelt, K. M., Aach, J., Guell, M., DiCarlo, J. E., et al. (2013). RNA-guided human genome engineering via Cas9. Science 339 (6121), 823–826. doi:10.1126/science.1232033
Malnoy, M., Viola, R., Jung, M. H., Koo, O. J., Kim, S., Kim, J. S., et al. (2016). DNA-free genetically edited grapevine and apple protoplast using CRISPR/Cas9 ribonucleoproteins. Front. Plant Sci. 7, 1904. doi:10.3389/fpls.2016.01904
Mangandi, J., Verma, S., Osorio, L., Peres, N. A., van de Weg, E., and Whitaker, V. M. (2017). Pedigree-based analysis in a multiparental population of octoploid strawberry reveals QTL alleles conferring resistance to phytophthora cactorum. G3 Genes|Genomes|Genetics 7 (6), 1707–1719. doi:10.1534/g3.117.042119
Mao, J., Wang, Y., Wang, B., Li, J., Zhang, C., Zhang, W., et al. (2023). High-quality haplotype-resolved genome assembly of cultivated octoploid strawberry. Hortic. Res. 10 (1), uhad002. doi:10.1093/hr/uhad002
Mao, W., Han, Y., Chen, Y., Sun, M., Feng, Q., Li, L., et al. (2022). Low temperature inhibits anthocyanin accumulation in strawberry fruit by activating FvMAPK3-induced phosphorylation of FvMYB10 and degradation of Chalcone Synthase 1. Plant Cell 34 (4), 1226–1249. doi:10.1093/plcell/koac006
Martín-Pizarro, C., Triviño, J. C., and Posé, D. (2019). Functional analysis of the TM6 MADS-box gene in the octoploid strawberry by CRISPR/Cas9-directed mutagenesis. J. Exp. Bot. 70 (3), 885–895. doi:10.1093/jxb/ery400
Martín-Pizarro, C., Vallarino, J. G., Osorio, S., Meco, V., Urrutia, M., Pillet, J., et al. (2021). The NAC transcription factor FaRIF controls fruit ripening in strawberry. Plant Cell 33 (5), 1574–1593. doi:10.1093/plcell/koab070
Martín-Trillo, M., Grandío, E. G., Serra, F., Marcel, F., Rodríguez-Buey, M. L., Schmitz, G., et al. (2011). Role of tomato BRANCHED1-like genes in the control of shoot branching. Plant J. 67 (4), 701–714. doi:10.1111/j.1365-313X.2011.04629.x
Mathews, H., Wagoner, W., Kellogg, J., and Bestwick, R. (1995). Genetic transformation of strawberry: stable integration of a gene to control biosynthesis of ethylene. Vitro Cell. Dev. Biol. - Plant 31 (1), 36–43. doi:10.1007/BF02632224
May, D., Paldi, K., and Altpeter, F. (2023). Targeted mutagenesis with sequence-specific nucleases for accelerated improvement of polyploid crops: progress, challenges, and prospects. Plant Genome 16 (2), e20298. doi:10.1002/tpg2.20298
Menz, J., Modrzejewski, D., Hartung, F., Wilhelm, R., and Sprink, T. (2020). Genome edited crops touch the market: a view on the global development and regulatory environment. Front. Plant Sci. 11, 586027. doi:10.3389/fpls.2020.586027
Menzel, C. (2021). Higher temperatures decrease fruit size in strawberry growing in the subtropics. Horticulturae 7 (2), 34–14. doi:10.3390/horticulturae7020034
Menzel, C. M. (2019). Temperature has a greater effect on fruit growth than defoliation or fruit thinning in strawberries in the subtropics. Agric. Switz. 9 (6), 127. doi:10.3390/agriculture9060127
Meuwissen, T. H. E., Hayes, B. J., and Goddard, M. E. (2001). Prediction of total genetic value using genome-wide dense marker maps. Available at: https://academic.oup.com/genetics/article/157/4/1819/6048353.
Miller, S. (2019). Gene editing of red raspberry (rubus ideaus L.) with CRISPR/Cas9 knocking out F3’H. Ås, Norway: Norwegian University of Life Sciences.
Min, K., Yi, G., Lee, J. G., Kim, H. S., Hong, Y., Choi, J. H., et al. (2020). Comparative transcriptome and metabolome analyses of two strawberry cultivars with different storability. PLoS ONE 15, e0242556. doi:10.1371/journal.pone.0242556
Mishra, R., Joshi, R. K., and Zhao, K. (2020). Base editing in crops: current advances, limitations and future implications. Plant Biotechnol. J. 18 (1), 20–31. doi:10.1111/pbi.13225
Miyawaki, K., Fukuoka, S., Kadomura, Y., Hamaoka, H., Mito, T., Ohuchi, H., et al. (2012). Establishment of a novel system to elucidate the mechanisms underlying light-induced ripening of strawberry fruit with an Agrobacterium-mediated RNAi technique. Plant Biotechnol. 29 (3), 271–277. doi:10.5511/plantbiotechnology.12.0406a
Molla, K. A., Sretenovic, S., Bansal, K. C., and Qi, Y. (2021). Precise plant genome editing using base editors and prime editors. Nat. Plants 7 (9), 1166–1187. doi:10.1038/s41477-021-00991-1
Montesinos-López, O. A., Montesinos-López, A., Pérez-Rodríguez, P., Barrón-López, J. A., Martini, J. W. R., Fajardo-Flores, S. B., et al. (2021). A review of deep learning applications for genomic selection. BMC Genomics 22 (1), 19. doi:10.1186/s12864-020-07319-x
Mouhu, K., Kurokura, T., Koskela, E. A., Albert, V. A., Elomaa, P., and Hytönen, T. (2013). The Fragaria vesca homolog of SUPPRESSOR OF OVEREXPRESSION OF CONSTANS1 represses flowering and promotes vegetative growth. Plant Cell 25 (9), 3296–3310. doi:10.1105/tpc.113.115055
Moyano-Cañete, E., Bellido, M. L., García-Caparrós, N., Medina-Puche, L., Amil-Ruiz, F., González-Reyes, J. A., et al. (2013). FaGAST2, a strawberry ripening-related gene, acts together with FaGAST1 to determine cell size of the fruit receptacle. Plant Cell Physiology 54 (2), 218–236. doi:10.1093/pcp/pcs167
Nagamatsu, S., Tsubone, M., Wada, T., Oku, K., Mori, M., Hirata, C., et al. (2021). Strawberry fruit shape: quantification by image analysis and qtl detection by genome-wide association analysis. Breed. Sci. 71 (2), 167–175. doi:10.1270/jsbbs.19106
Najafi, S., Bertini, E., D’Incà, E., Fasoli, M., and Zenoni, S. (2023). DNA-free genome editing in grapevine using CRISPR/Cas9 ribonucleoprotein complexes followed by protoplast regeneration. Hortic. Res. 10 (1), uhac240. doi:10.1093/hr/uhac240
Nehra, N. S., Chibbar, R. N., Kartha, K. K., Datla, R. S. S., Crosby, W. L., and Stushnoff, C. (1990a). Agrobacterium-mediated transformation of strawberry calli and recovery of transgenic plants. Plant Cell Rep. 9 (1), 10–13. doi:10.1007/BF00232125
Nehra, N. S., Chibbar, R. N., Kartha, K. K., Datla, R. S. S., Crosby, W. L., and Stushnoff, C. (1990b). Genetic transformation of strawberry by Agrobacterium tumefaciens using a leaf disk regeneration system. Plant Cell Rep. 9 (6), 293–298. doi:10.1007/BF00232854
Nellist, C. F., Vickerstaff, R. J., Sobczyk, M. K., Marina-Montes, C., Wilson, F. M., Simpson, D. W., et al. (2019). Quantitative trait loci controlling Phytophthora cactorum resistance in the cultivated octoploid strawberry (Fragaria × ananassa). Hortic. Res. 6 (1), 60. doi:10.1038/s41438-019-0136-4
Nelson, J. R., Verma, S., Bassil, N. V., Finn, C. E., Hancock, J. F., Cole, G. S., et al. (2021). Discovery of three loci increasing resistance to charcoal rot caused by macrophomina phaseolina in octoploid strawberry. G3 Genes, Genomes, Genet. 11 (3), jkab037. doi:10.1093/G3JOURNAL/JKAB037
Noh, Y. H., Lee, S., Whitaker, V. M., Cearley, K. R., and Cha, J. S. (2017). A high-throughput marker-assisted selection system combining rapid DNA extraction high-resolution melting and simple sequence repeat analysis: strawberry as a model for fruit crops. J. Berry Res. 7 (1), 23–31. doi:10.3233/JBR-160145
Nyman, M., and Wallin, A. (1988). Plant regeneration from strawberry (fragaria x ananassa) mesophyll protoplasts. J. Plant Physiology 133 (3), 375–377. doi:10.1016/S0176-1617(88)80220-7
Nyman, M., and Wallin, A. (1992a). Improved culture technique for strawberry (Fragaria FFFD ananassa Duch.) protoplasts and the determination of DNA content in protoplast derived plants. Plant Cell, Tissue Organ Cult. 30 (2), 127–133. doi:10.1007/BF00034306
Nyman, M., and Wallin, A. (1992b). Transient gene expression in strawberry (Fragaria x ananassa Duch.) protoplasts and the recovery of transgenic plants. Plant Cell Rep. 11 (2), 105–108. doi:10.1007/BF00235264
Oh, Y., Barbey, C. R., Chandra, S., Bai, J., Fan, Z., Plotto, A., et al. (2021). Genomic characterization of the fruity aroma gene, FaFAD1, reveals a gene dosage effect on γ-decalactone production in strawberry (fragaria × ananassa). Front. Plant Sci. 12, 639345. doi:10.3389/fpls.2021.639345
Oh, Y., Zurn, J. D., Bassil, N., Edger, P. P., Knapp, S. J., Whitaker, V. M., et al. (2019). The strawberry DNA testing handbook. HortScience Horts 54 (12), 2267–2270. doi:10.21273/HORTSCI14387-19
Oosumi, T., Gruszewski, H. A., Blischak, L. A., Baxter, A. J., Wadl, P. A., Shuman, J. L., et al. (2006). High-efficiency transformation of the diploid strawberry (Fragaria vesca) for functional genomics. Planta 223 (6), 1219–1230. doi:10.1007/s00425-005-0170-3
Osakabe, Y., Liang, Z., Ren, C., Nishitani, C., Osakabe, K., Wada, M., et al. (2018). CRISPR–Cas9-mediated genome editing in apple and grapevine. Nat. Protoc. 13 (12), 2844–2863. doi:10.1038/s41596-018-0067-9
Osorio, L. F., Gezan, S. A., Verma, S., and Whitaker, V. M. (2021). Independent validation of genomic prediction in strawberry over multiple cycles. Front. Genet. 11, 596258. doi:10.3389/fgene.2020.596258
Oz, M. T., Altpeter, A., Karan, R., Merotto, A., and Altpeter, F. (2021). CRISPR/Cas9-Mediated multi-allelic gene targeting in sugarcane confers herbicide tolerance. Front. Genome Ed. 3, 673566. doi:10.3389/fgeed.2021.673566
Ozyigit, I. I., Dogan, I., and Artam Tarhan, E. (2013). “Agrobacterium rhizogenes-mediated transformation and its biotechnological applications in crops,” in Crop improvement: new approaches and modern techniques. Editors K. R. Hakeem, P. Ahmad, and M. Ozturk (Springer US), 1–48. doi:10.1007/978-1-4614-7028-1_1
Paine, J. A., Shipton, C. A., Chaggar, S., Howells, R. M., Kennedy, M. J., Vernon, G., et al. (2005). Improving the nutritional value of Golden Rice through increased pro-vitamin A content. Nat. Biotechnol. 23 (4), 482–487. doi:10.1038/nbt1082
Pan, C., Sretenovic, S., and Qi, Y. (2021). CRISPR/dCas-mediated transcriptional and epigenetic regulation in plants. Curr. Opin. Plant Biol. 60, 101980. doi:10.1016/j.pbi.2020.101980
Pang, H. G., Yan, Q., Zhao, S., He, F., Xu, J. F., Qi, B. X., et al. (2019). Knockout of the S-acyltransferase gene, pbpat14, confers the dwarf yellowing phenotype in first generation pear by ABA accumulation. Int. J. Mol. Sci. 20 (24), 6347. doi:10.3390/ijms20246347
Paniagua, C., Blanco-Portales, R., Barceló-Muñoz, M., García-Gago, J. A., Waldron, K. W., Quesada, M. A., et al. (2016). Antisense down-regulation of the strawberry β-galactosidase gene FaβGal4 increases cell wall galactose levels and reduces fruit softening. J. Exp. Bot. 67 (3), 619–631. doi:10.1093/jxb/erv462
Paniagua, C., Ric-Varas, P., García-Gago, J. A., López-Casado, G., Blanco-Portales, R., Muñoz-Blanco, J., et al. (2020). Elucidating the role of polygalacturonase genes in strawberry fruit softening. J. Exp. Bot. 71 (22), 7103–7117. doi:10.1093/jxb/eraa398
Paniagua, C., Sánchez-Raya, C., Blanco-Portales, R., Mercado, J. A., Palomo-Ríos, E., and Posé, S. (2022). Silencing of FaPG1, a fruit specific polygalacturonase gene. Decreased Strawb. Fruit. Fungal Decay Dur. Postharvest 96. doi:10.3390/iecps2021-12049
Pantazis, C. J., Fisk, S., Mills, K., Flinn, B. S., Shulaev, V., Veilleux, R. E., et al. (2013). Development of an efficient transformation method by Agrobacterium tumefaciens and high throughput spray assay to identify transgenic plants for woodland strawberry (Fragaria vesca) using NPTII selection. Plant Cell Rep. 32 (3), 329–337. doi:10.1007/s00299-012-1366-1
Pattanaik, S., Dey, N., Bhattacharyya, S., and Maiti, I. B. (2004). Isolation of full-length transcript promoter from the Strawberry vein banding virus (SVBV) and expression analysis by protoplasts transient assays and in transgenic plants. Plant Sci. 167 (3), 427–438. doi:10.1016/j.plantsci.2004.04.011
Perk, E. A., Arruebarrena Di Palma, A., Colman, S., Mariani, O., Cerrudo, I., D’Ambrosio, J. M., et al. (2023). CRISPR/Cas9-mediated phospholipase C 2 knock-out tomato plants are more resistant to Botrytis cinerea. Planta 257 (6), 117. doi:10.1007/s00425-023-04147-7
Perkins-Veazie, P. (1995). “Growth and ripening of strawberry fruit,” in Horticultural reviews, 267–297. doi:10.1002/9780470650585.ch8
Petrasch, S., Knapp, S. J., van Kan, J. A. L., and Blanco-Ulate, B. (2019). Grey mould of strawberry, a devastating disease caused by the ubiquitous necrotrophic fungal pathogen Botrytis cinerea. Mol. Plant Pathol. 20 (6), 877–892. doi:10.1111/mpp.12794
Pi, M., Hu, S., Cheng, L., Zhong, R., Cai, Z., Liu, Z., et al. (2021). The MADS-box gene FveSEP3 plays essential roles in flower organogenesis and fruit development in woodland strawberry. Hortic. Res. 8 (1), 247. doi:10.1038/s41438-021-00673-1
Pillet, J., Chambers, A. H., Barbey, C., Bao, Z., Plotto, A., Bai, J., et al. (2017). Identification of a methyltransferase catalyzing the final step of methyl anthranilate synthesis in cultivated strawberry. BMC Plant Biol. 17 (1), 147. doi:10.1186/s12870-017-1088-1
Pincot, D. D. A., Hardigan, M. A., Cole, G. S., Famula, R. A., Henry, P. M., Gordon, T. R., et al. (2020). Accuracy of genomic selection and long-term genetic gain for resistance to Verticillium wilt in strawberry. Plant Genome 13 (3), e20054. doi:10.1002/tpg2.20054
Pincot, D. D. A., Poorten, T. J., Hardigan, M. A., Harshman, J. M., Acharya, C. B., Cole, G. S., et al. (2018). Genome-wide association mapping uncovers Fw1, a dominant gene conferring resistance to Fusarium wilt in strawberry. G3 Genes, Genomes, Genet. 8 (5), 1817–1828. doi:10.1534/g3.118.200129
Pompili, V., Dalla Costa, L., Piazza, S., Pindo, M., and Malnoy, M. (2020). Reduced fire blight susceptibility in apple cultivars using a high-efficiency CRISPR/Cas9-FLP/FRT-based gene editing system. Plant Biotechnol. J. 18 (3), 845–858. doi:10.1111/pbi.13253
Porter, M., Fan, Z., Lee, S., and Whitaker, V. M. (2023). Strawberry breeding for improved flavor. Crop Sci. 63 (4), 1949–1963. doi:10.1002/csc2.21012
Posé, S., Paniagua, C., Cifuentes, M., Blanco-Portales, R., Quesada, M. A., and Mercado, J. A. (2013). Insights into the effects of polygalacturonase FaPG1 gene silencing on pectin matrix disassembly, enhanced tissue integrity, and firmness in ripe strawberry fruits. J. Exp. Bot. 64 (12), 3803–3815. doi:10.1093/jxb/ert210
Potter, D., Eriksson, T., Evans, R. C., Oh, S., Smedmark, J. E. E., Morgan, D. R., et al. (2007). Phylogeny and classification of Rosaceae. Plant Syst. Evol. 266 (1), 5–43. doi:10.1007/s00606-007-0539-9
Potter, D., Gao, F., Bortiri, P. E., Oh, S.-H., and Baggett, S. (2002). Phylogenetic relationships in Rosaceae inferred from chloroplast matK and trnL-trnF nucleotide sequence data. Plant Syst. Evol. 231 (1), 77–89. doi:10.1007/s006060200012
Premsekhar, M., and Rajashree, V. (2009). V: influence of bio-fertilizers on the growth characters, yield attributes, yield and quality of tomato: Am. J. Sustain. Agric. 3 (1), 68–70.
Prías-Blanco, M., Chappell, T. M., Freed, E. F., Illa-Berenguer, E., Eckert, C. A., and Parrott, W. A. (2022). An Agrobacterium strain auxotrophic for methionine is useful for switchgrass transformation. Transgenic Res. 31 (6), 661–676. doi:10.1007/s11248-022-00328-4
Qi, X., Liu, C., Song, L., and Li, M. (2020). PaMADS7, a MADS-box transcription factor, regulates sweet cherry fruit ripening and softening. Plant Sci. 301, 110634. doi:10.1016/j.plantsci.2020.110634
Qiao, Q., Edger, P. P., Xue, L., Qiong, L., Lu, J., Zhang, Y., et al. (2021). Evolutionary history and pan-genome dynamics of strawberry (Fragaria spp.). Proc. Natl. Acad. Sci. 118 (45), e2105431118. doi:10.1073/pnas.2105431118
Qiu, Y., Guan, S. C., Wen, C., Li, P., Gao, Z., and Chen, X. (2019). Auxin and cytokinin coordinate the dormancy and outgrowth of axillary bud in strawberry runner. BMC Plant Biol. 19 (1), 528. doi:10.1186/s12870-019-2151-x
Quarshi, H. Q., Ahmed, W., Azmant, R., Chendouh-Brahmi, N., Quyyum, A., and Abbas, A. (2023). “Post-harvest problems of strawberry and their solutions,” in Recent studies on strawberries. Editor N. E. Kafkas (London, United Kingdom: IntechOpen). doi:10.5772/intechopen.102963
Quesada, M. A., Blanco-Portales, R., Posé, S., García-Gago, J. A., Jiménez-Bermúdez, S., Muñoz-Serrano, A., et al. (2009). Antisense down-regulation of the FaPG1 gene reveals an unexpected central role for polygalacturonase in strawberry fruit softening1[W]. Plant Physiol. 150 (2), 1022–1032. doi:10.1104/pp.109.138297
Raab, T., López-Ráez, J. A., Klein, D., Caballero, J. L., Moyano, E., Schwab, W., et al. (2006). FaQR, required for the biosynthesis of the strawberry flavor compound 4-hydroxy-2,5-dimethyl-3(2H)-Furanone, encodes an enone oxidoreductase. Plant Cell 18 (4), 1023–1037. doi:10.1105/tpc.105.039784
Reintanz, B., Lehnen, M., Reichelt, M., Gershenzon, J., Kowalczyk, M., Sandberg, G., et al. (2001). Bus, a bushy Arabidopsis CYP79F1 knockout mutant with abolished synthesis of short-chain aliphatic glucosinolates. Plant Cell 13 (2), 351–367. doi:10.1105/tpc.13.2.351
Rey-Serra, P., Mnejja, M., and Monfort, A. (2021). Shape, firmness and fruit quality QTLs shared in two non-related strawberry populations. Plant Sci. 311, 111010. doi:10.1016/j.plantsci.2021.111010
Saiga, S., Tada, M., Segawa, T., Sugihara, Y., Nishikawa, M., Makita, N., et al. (2022). NGS-based genome wide association study helps to develop co-dominant marker for the physical map-based locus of PFRU controlling flowering in cultivated octoploid strawberry. Euphytica 219 (1), 6. doi:10.1007/s10681-022-03132-7
Saint-Oyant, L. H., Ruttink, T., Hamama, L., Kirov, I., Lakhwani, D., Zhou, N. N., et al. (2018). A high-quality genome sequence of Rosa chinensis to elucidate ornamental traits. Nat. Plants 4 (7), 473–484. doi:10.1038/s41477-018-0166-1
Sakamoto, T., and Matsuoka, M. (2004). Generating high-yielding varieties by genetic manipulation of plant architecture. Curr. Opin. Biotechnol. 15 (2), 144–147. doi:10.1016/j.copbio.2004.02.003
Salinas, N., Verma, S., Peres, N., and Whitaker, V. M. (2019). FaRCa1: a major subgenome-specific locus conferring resistance to Colletotrichum acutatum in strawberry. Theor. Appl. Genet. 132 (4), 1109–1120. doi:10.1007/s00122-018-3263-7
Sánchez-Sevilla, J. F., Cruz-Rus, E., Valpuesta, V., Botella, M. A., and Amaya, I. (2014). Deciphering gamma-decalactone biosynthesis in strawberry fruit using a combination of genetic mapping, RNA-Seq and eQTL analyses. BMC Genomics 15 (1), 218. doi:10.1186/1471-2164-15-218
Sánchez-Sevilla, J. F., Vallarino, J. G., Osorio, S., Bombarely, A., Posé, D., Merchante, C., et al. (2017). Gene expression atlas of fruit ripening and transcriptome assembly from RNA-seq data in octoploid strawberry (Fragaria × ananassa). Sci. Rep. 7 (1), 13737. doi:10.1038/s41598-017-14239-6
Sasaki, Y., Orikasa, T., Nakamura, N., Hayashi, K., Yasaka, Y., Makino, N., et al. (2022). Optimal packaging for strawberry transportation: evaluation and modeling of the relationship between food loss reduction and environmental impact. J. Food Eng. 314, 110767. doi:10.1016/j.jfoodeng.2021.110767
Schaart, J. G. (2014). Agrobacterium-mediated transformation of strawberry. Iss 4. doi:10.21769/bioprotoc.1022
Schestibratov, K. A., and Dolgov, S. V. (2005). Transgenic strawberry plants expressing a thaumatin II gene demonstrate enhanced resistance to Botrytis cinerea. Sci. Hortic. 106 (2), 177–189. doi:10.1016/j.scienta.2005.03.016
Schwab, W., Davidovich-Rikanati, R., and Lewinsohn, E. (2008). Biosynthesis of plant-derived flavor compounds. Plant J. 54 (4), 712–732. doi:10.1111/j.1365-313X.2008.03446.x
Session, A. M., and Rokhsar, D. S. (2023). Transposon signatures of allopolyploid genome evolution. Nat. Commun. 14 (1), 3180. doi:10.1038/s41467-023-38560-z
Shanmugam, A., Hossain, M. R., Natarajan, S., Jung, H.-J., Song, J.-Y., Kim, H.-T., et al. (2017). Sugar content analysis and expression profiling of sugar related genes in contrasting Strawberry (Fragaria × ananassa) cultivars. J. Plant Biotechnol. 44 (2), 178–190. doi:10.5010/JPB.2017.44.2.178
Sharma, R. R., and Singh, R. (2009). Gibberellic acid influences the production of malformed and button berries, and fruit yield and quality in strawberry (Fragaria × ananassa Duch.). Sci. Hortic. 119 (4), 430–433. doi:10.1016/j.scienta.2008.11.002
Shen, B., Zhang, W., Zhang, J., Zhou, J., Wang, J., Chen, L., et al. (2014). Efficient genome modification by CRISPR-Cas9 nickase with minimal off-target effects. Nat. Methods 11 (4), 399–402. doi:10.1038/nmeth.2857
Shi, J., Gao, H., Wang, H., Lafitte, H. R., Archibald, R. L., Yang, M., et al. (2017). ARGOS8 variants generated by CRISPR-Cas9 improve maize grain yield under field drought stress conditions. Plant Biotechnol. J. 15 (2), 207–216. doi:10.1111/pbi.12603
Shirasawa, K., Hirakawa, H., Nakayama, S., Sasamoto, S., Tsuruoka, H., Minami, C., et al. (2021). A chromosome-scale strawberry genome assembly of a Japanese variety, Reikou. BioRxiv. doi:10.1101/2021.04.23.441065
Shu, P., Li, Z., Min, D., Zhang, X., Ai, W., Li, J., et al. (2020). CRISPR/Cas9-Mediated SlMYC2 mutagenesis adverse to tomato plant growth and MeJA-induced fruit resistance to Botrytis cinerea. J. Agric. Food Chem. 68 (20), 5529–5538. doi:10.1021/acs.jafc.9b08069
Shulaev, V., Korban, S. S., Sosinski, B., Abbott, A. G., Aldwinckle, H. S., Folta, K. M., et al. (2008). Multiple models for Rosaceae genomics. Plant Physiol. 147 (3), 985–1003. doi:10.1104/pp.107.115618
Shulaev, V., Sargent, D. J., Crowhurst, R. N., Mockler, T. C., Folkerts, O., Delcher, A. L., et al. (2011). The genome of woodland strawberry (Fragaria vesca). Nat. Genet. 43 (2), 109–116. doi:10.1038/ng.740
Singh, A., and Roychoudhury, A. (2023). “RNA interference (RNAi) technology: an effective tool in plant breeding,” in Advanced crop improvement, volume 1: theory and practice. Editors A. Raina, M. R. Wani, R. A. Laskar, N. Tomlekova, and S. Khan (Springer International Publishing), 309–320. doi:10.1007/978-3-031-28146-4_12
Song, Y., Li, C., Liu, L., Hu, P., Li, G., Zhao, X., et al. (2023a). The population genomic analyses of chloroplast genomes shed new insights on the complicated ploidy and evolutionary history in Fragaria. Front. Plant Sci. 13, 1065218. doi:10.3389/fpls.2022.1065218
Song, Y., Peng, Y., Liu, L., Li, G., Zhao, X., Wang, X., et al. (2023b). Phased gap-free genome assembly of octoploid cultivated strawberry illustrates the genetic and epigenetic divergence among subgenomes. Hortic. Res. 11, uhad252. doi:10.1093/hr/uhad252
Sønsteby, A., Woznicki, T. L., and Heide, O. M. (2021). Effects of runner removal and partial defoliation on the growth and yield performance of ‘Favori’ everbearing strawberry plants. Horticulturae 7 (8), 215. doi:10.3390/horticulturae7080215
Soyk, S., Müller, N. A., Park, S. J., Schmalenbach, I., Jiang, K., Hayama, R., et al. (2017). Variation in the flowering gene SELF PRUNING 5G promotes day-neutrality and early yield in tomato. Nat. Genet. 49 (1), 162–168. doi:10.1038/ng.3733
Srivastava, K. K., Kumar, D., Singh, S. R., Chand, O., and Pandey, S. (2019). Effect of tree architecture and variety on growth and yield attributes of apple under UHDP. J. Crop Weed 15 (Issue 1).
Stirnberg, P., van de Sande, K., and Leyser, H. M. O. (2002). MAX1 and MAX2 control shoot lateral branching in Arabidopsis. Development 129 (5), 1131–1141. doi:10.1242/dev.129.5.1131
Stokstad, E. (2024). In Antarctica, scientists track a dangerous bird flu. Science 383, 1281. doi:10.1126/science.adp3271
Su, L., Bassa, C., Audran, C., Mila, I., Cheniclet, C., Chevalier, C., et al. (2014). The auxin sl-IAA17 transcriptional repressor controls fruit size via the regulation of endoreduplication-related cell expansion. Plant Cell Physiology 55 (11), 1969–1976. doi:10.1093/pcp/pcu124
Sukegawa, S., Nureki, O., Toki, S., and Saika, H. (2023). Genome editing in rice mediated by miniature size Cas nuclease SpCas12f. Front. Genome Ed. 5, 1138843. doi:10.3389/fgeed.2023.1138843
Sun, J.-H., Luo, J.-J., Tian, L., Li, C.-L., Xing, Y., and Shen, Y.-Y. (2013). New evidence for the role of ethylene in strawberry fruit ripening. J. Plant Growth Regul. 32 (3), 461–470. doi:10.1007/s00344-012-9312-6
Symons, G. M., Chua, Y. J., Ross, J. J., Quittenden, L. J., Davies, N. W., and Reid, J. B. (2012). Hormonal changes during non-climacteric ripening in strawberry. J. Exp. Bot. 63 (13), 4741–4750. doi:10.1093/jxb/ers147
Tang, D., Jia, Y., Zhang, J., Li, H., Cheng, L., Wang, P., et al. (2022). Genome evolution and diversity of wild and cultivated potatoes. Nature 606 (7914), 535–541. doi:10.1038/s41586-022-04822-x
Tantikanjana, T., Yong, J. W. H., Letham, D. S., Griffith, M., Hussain, M., Ljung, K., et al. (2001). Control of axillary bud initiation and shoot architecture in Arabidopsis through the SUPERSHOOT gene. Genes Dev. 15 (12), 1577–1588. doi:10.1101/gad.887301
Tao, Y., Zhao, X., Mace, E., Henry, R., and Jordan, D. (2019). Exploring and exploiting pan-genomics for crop improvement. Mol. Plant 12 (2), 156–169. doi:10.1016/j.molp.2018.12.016
Tennessen, J. A., Govindarajulu, R., Ashman, T.-L., and Liston, A. (2014). Evolutionary origins and dynamics of octoploid strawberry subgenomes revealed by dense targeted capture linkage maps. Genome Biol. Evol. 6 (12), 3295–3313. doi:10.1093/gbe/evu261
Tenreira, T., Pimenta Lange, M. J., Lange, T., Bres, C., Labadie, M., Monfort, A., et al. (2017). A specific gibberellin 20-oxidase dictates the flowering-runnering decision in diploid strawberry. Plant Cell 29 (9), 2168–2182. doi:10.1105/tpc.16.00949
Thompson, P. A., and Guttridge, C. G. (1959). Effect of gibberellic acid on the initiation of flowers and runners in the strawberry. Nature 184 (4688), BA72–BA73. doi:10.1038/184072a0b
Tian, J., Cheng, L., Han, Z., and Yao, Y. (2015). Tobacco rattle virus mediated gene silencing in strawberry plants. Plant Cell, Tissue Organ Cult. (PCTOC) 120 (3), 1131–1138. doi:10.1007/s11240-014-0669-z
Trevino, A. E., and Zhang, F. (2014). “Chapter eight - genome editing using Cas9 nickases,” in Methods in enzymology. Editors J. A. Doudna, and E. J. Sontheimer (Academic Press), 546, 161–174. doi:10.1016/B978-0-12-801185-0.00008-8
Tsai, S. Q., Wyvekens, N., Khayter, C., Foden, J. A., Thapar, V., Reyon, D., et al. (2014). Dimeric CRISPR RNA-guided FokI nucleases for highly specific genome editing. Nat. Biotechnol. 32 (6), 569–576. doi:10.1038/nbt.2908
Tulipani, S., Mezzetti, B., Capocasa, F., Bompadre, S., Beekwilder, J., de Vos, C. H. R., et al. (2008). Antioxidants, phenolic compounds, and nutritional quality of different strawberry genotypes. J. Agric. Food Chem. 56 (3), 696–704. doi:10.1021/jf0719959
Tuncel, A., and Qi, Y. (2022). CRISPR/Cas mediated genome editing in potato: past achievements and future directions. Plant Sci. 325, 111474. doi:10.1016/j.plantsci.2022.111474
Turner, J. N. (1963). Application of gibberellic acid to strawberry plants at different stages of development. Nature 197 (4862), 95–96. doi:10.1038/197095b0
Ulrich, D., Komes, D., Olbricht, K., and Hoberg, E. (2007). Diversity of aroma patterns in wild and cultivated Fragaria accessions. Genet. Resour. Crop Evol. 54 (6), 1185–1196. doi:10.1007/s10722-006-9009-4
United States Department of Agriculture (2022). History highlight: Aphis begins overseeing AG biotech. United States department of agriculture, animal and plant health inspection Service. Available at: https://www.aphis.usda.gov/aphis/newsroom/stakeholder-info/sa_by_date/sa-2022/aphis50-hh-biotech#:∼:text=APHIS’%20first%20major%20biotechnology%20regulation,used%20in%20the%20creation%20process.
United States Food and Drug Administration (2024). New plant variety regulatory information. U.S Food and Drug Administration. Available at: https://www.fda.gov/food/food-new-plant-varieties/new-plant-variety-regulatory-information#:∼:text=The%20FDA%20regulates%20%20human%20and.sold%20in%20the%20the%20United%20states.
Vallarino, J. G., de Abreu e Lima, F., Soria, C., Tong, H., Pott, D. M., Willmitzer, L., et al. (2018). Genetic diversity of strawberry germplasm using metabolomic biomarkers. Sci. Rep. 8 (1), 14386. doi:10.1038/s41598-018-32212-9
Vallarino, J. G., Pott, D. M., Cruz-Rus, E., Miranda, L., Medina-Minguez, J. J., Valpuesta, V., et al. (2019). Identification of quantitative trait loci and candidate genes for primary metabolite content in strawberry fruit. Hortic. Res. 6 (1), 4. doi:10.1038/s41438-018-0077-3
Varshney, R. K., Terauchi, R., and Mccouch, S. R. (2014). Harvesting the promising fruits of genomics: applying genome sequencing technologies to crop breeding. PLOS Biol. 12, e1001883. doi:10.1371/journal.pbio.1001883
Vellicce, G. R., Coll, Y., Castagnaro, A. P., Ricci, D., and Carlos, J. (2003). Transformation of a strawberry cultivar using a modified regeneration medium. HORTSCIENCE 38 (Issue 2), 277–280. doi:10.21273/hortsci.38.2.277
Verma, S., Zurn, J. D., Salinas, N., Mathey, M. M., Denoyes, B., Hancock, J. F., et al. (2017). Clarifying sub-genomic positions of QTLs for flowering habit and fruit quality in U.S. strawberry (Fragaria×ananassa) breeding populations using pedigree-based QTL analysis. Hortic. Res. 4, 17062. doi:10.1038/hortres.2017.62
Vigouroux, A., and Bikard, D. (2020). CRISPR tools to control gene expression in bacteria. Microbiol. Mol. Biol. Rev. 84 (2), 000777–e119. doi:10.1128/mmbr.00077-19
Vilanova, S., Sargent, D. J., Arús, P., and Monfort, A. (2008). Synteny conservation between two distantly-related Rosaceae genomes: Prunus (the stone fruits) and Fragaria(the strawberry). BMC Plant Biol. 8 (1), 67. doi:10.1186/1471-2229-8-67
Vo, P. L. H., Ronda, C., Klompe, S. E., Chen, E. E., Acree, C., Wang, H. H., et al. (2021). CRISPR RNA-guided integrases for high-efficiency, multiplexed bacterial genome engineering. Nat. Biotechnol. 39 (4), 480–489. doi:10.1038/s41587-020-00745-y
Vu, K. D., Hollingsworth, R. G., Leroux, E., Salmieri, S., and Lacroix, M. (2011). Development of edible bioactive coating based on modified chitosan for increasing the shelf life of strawberries. Food Res. Int. 44 (1), 198–203. doi:10.1016/j.foodres.2010.10.037
Wada, T., Tsubone, M., Mori, M., Hirata, C., Nagamatsu, S., Oku, K., et al. (2020). Genome-wide association study of strawberry fruit quality-related traits using a magic population derived from crosses involving six strawberry cultivars. Hortic. J. 89 (5), 553–566. doi:10.2503/hortj.UTD-180
Waltz, E. (2016). Gene-edited CRISPR mushroom escapes US regulation. Nature 532 (7599), 293. doi:10.1038/nature.2016.19754
Wang, B., Li, N., Huang, S., Hu, J., Wang, Q., Tang, Y., et al. (2021a). Enhanced soluble sugar content in tomato fruit using CRISPR/Cas9-mediated SlINVINH1 and SlVPE5 gene editing. PeerJ 9, e12478. doi:10.7717/peerj.12478
Wang, D., Samsulrizal, N. H., Yan, C., Allcock, N. S., Craigon, J., Blanco-Ulate, B., et al. (2019). Characterization of CRISPR mutants targeting genes modulating pectin degradation in ripening tomato. Plant Physiol. 179 (2), 544–557. doi:10.1104/pp.18.01187
Wang, H., Zhang, H., Yang, Y., Li, M., Zhang, Y., Liu, J., et al. (2020a). The control of red colour by a family of MYB transcription factors in octoploid strawberry (Fragaria × ananassa) fruits. Plant Biotechnol. J. 18 (5), 1169–1184. doi:10.1111/pbi.13282
Wang, J., Ge, H., Peng, S., Zhang, H., Chen, P., and Xu, J. (2004). Transformation of strawberry (Fragaria ananassa Duch.) with late embryogenesis abundant protein gene. J. Hortic. Sci. Biotechnol. 79 (5), 735–738. doi:10.1080/14620316.2004.11511835
Wang, M., Lu, Y., Botella, J. R., Mao, Y., Hua, K., and Zhu, J. kang. (2017). Gene targeting by homology-directed repair in rice using a geminivirus-based CRISPR/Cas9 system. Mol. Plant 10 (7), 1007–1010. doi:10.1016/j.molp.2017.03.002
Wang, M., Xu, Z., Gosavi, G., Ren, B., Cao, Y., Kuang, Y., et al. (2020b). Targeted base editing in rice with CRISPR/ScCas9 system. Plant Biotechnol. J. 18 (8), 1645–1647. doi:10.1111/pbi.13330
Wang, S., Song, M., Guo, J., Huang, Y., Zhang, F., Xu, C., et al. (2018). The potassium channel FaTPK1 plays a critical role in fruit quality formation in strawberry (Fragaria × ananassa). Plant Biotechnol. J. 16 (3), 737–748. doi:10.1111/pbi.12824
Wang, Y., Kong, L., Wang, W., and Qin, G. (2023a). Global ubiquitinome analysis reveals the role of E3 ubiquitin ligase FaBRIZ in strawberry fruit ripening. J. Exp. Bot. 74 (1), 214–232. doi:10.1093/jxb/erac400
Wang, Y., Yu, J., Jiang, M., Lei, W., Zhang, X., and Tang, H. (2023b). “Sequencing and assembly of polyploid genomes,” in Polyploidy: methods and protocols. Editor Y. Van de Peer (Springer US), 429–458. doi:10.1007/978-1-0716-2561-3_23
Wang, Y., Zhao, F., Zhang, G., Jia, S., and Yan, Z. (2021b). FaWRKY11 transcription factor positively regulates resistance to Botrytis cinerea in strawberry fruit. Sci. Hortic. 279, 109893. doi:10.1016/j.scienta.2020.109893
Wang, Z., Ma, Y., Chen, M., Da, L., Su, Z., Zhang, Z., et al. (2023c). Comparative genomics analysis of WAK/WAKL family in Rosaceae identify candidate WAKs involved in the resistance to Botrytis cinerea. BMC Genomics 24 (1), 337. doi:10.1186/s12864-023-09371-9
Weebadde, C. K., Wang, D., Finn, C. E., Lewers, K. S., Luby, J. J., Bushakra, J., et al. (2008). Using a linkage mapping approach to identify QTL for day-neutrality in the octoploid strawberry. Plant Breed. 127 (1), 94–101. doi:10.1111/j.1439-0523.2007.01430.x
Werner, C. R., Gaynor, R. C., Sargent, D. J., Lillo, A., Gorjanc, G., and Hickey, J. M. (2023). Genomic selection strategies for clonally propagated crops. Theor. Appl. Genet. 136 (4), 74. doi:10.1007/s00122-023-04300-6
Whitaker, V. M., Knapp, S. J., Hardigan, M. A., Edger, P. P., Slovin, J. P., Bassil, N. V., et al. (2020). A roadmap for research in octoploid strawberry. Hortic. Res. 7 (Issue 1), 33. doi:10.1038/s41438-020-0252-1
Whitaker, V. M., Lee, S., Osorio, L. F., Verma, S., Roach, J. A., Mangandi, J., et al. (2017). Advances in strawberry breeding at the University of Florida. Acta Hortic. 1156, 1–6. doi:10.17660/ActaHortic.2017.1156.1
Wilson, F. M., Harrison, K., Armitage, A. D., Simkin, A. J., and Harrison, R. J. (2019). CRISPR/Cas9-mediated mutagenesis of phytoene desaturase in diploid and octoploid strawberry. Plant Methods 15 (1), 45. doi:10.1186/s13007-019-0428-6
Wu, K.-L., Guo, Z.-J., Wang, H.-H., and Li, J. (2005a). The WRKY family of transcription factors in rice and Arabidopsis and their origins. DNA Res. 12 (1), 9–26. doi:10.1093/dnares/12.1.9
Xing, S., Chen, K., Zhu, H., Zhang, R., Zhang, H., Li, B., et al. (2020). Fine-tuning sugar content in strawberry. Genome Biol. 21 (1), 230. doi:10.1186/s13059-020-02146-5
Xing, S., Jia, M., Wei, L., Mao, W., Abbasi, U. A., Zhao, Y., et al. (2018). CRISPR/Cas9-introduced single and multiple mutagenesis in strawberry. J. Genet. Genomics 45 (12), 685–687. doi:10.1016/j.jgg.2018.04.006
Xing, S., Sun, Y., Li, B., Li, H., Zhao, K. T., Chen, K., et al. (2023). Anthocyanin-assisted <italic&gt;Agrobacterium&lt;/italic&gt; infiltration for the rapid evaluation of genome editing efficiencies across multiple plant species. Natl. Sci. Open 2 (5), 20220052. doi:10.1360/nso/20220052
Yamamoto, E., Kataoka, S., Shirasawa, K., Noguchi, Y., and Isobe, S. (2021). Genomic selection for F1 hybrid breeding in strawberry (fragaria × ananassa). Front. Plant Sci. 12, 645111. doi:10.3389/fpls.2021.645111
Yan, H., Ma, D., Yi, P., Sun, G., Chen, X., Yi, Y., et al. (2023). Highly efficient Agrobacterium rhizogenes-mediated transformation for functional analysis in woodland strawberry. Plant Methods 19 (1), 99. doi:10.1186/s13007-023-01078-y
Yi, G., Shin, H., Min, K., and Lee, E. J. (2021). Expanded transcriptomic view of strawberry fruit ripening through meta-analysis. PLoS ONE 16 (6 June), e0252685. doi:10.1371/journal.pone.0252685
Yu, Y. Y., Dou, G. X., Sun, X. X., Chen, L., Zheng, Y., Xiao, H. M., et al. (2021). Transcriptome and biochemical analysis jointly reveal the effects of Bacillus cereus AR156 on postharvest strawberry Gray Mold and fruit quality. Front. Plant Sci. 12, 700446. doi:10.3389/fpls.2021.700446
Yue, M., Jiang, L., Zhang, N., Zhang, L., Liu, Y., Wang, Y., et al. (2022). Importance of FaWRKY71 in strawberry (fragaria × ananassa) fruit ripening. Int. J. Mol. Sci. 23 (20), 12483. doi:10.3390/ijms232012483
Yuste-Lisbona, F. J., Fernández-Lozano, A., Pineda, B., Bretones, S., Ortíz-Atienza, A., García-Sogo, B., et al. (2020). ENO regulates tomato fruit size through the floral meristem development network. Proc. Natl. Acad. Sci. U. S. A. 117, 8187–8195. doi:10.1073/pnas.1913688117
Zabetakis, I., and Holden, M. A. (1997). Strawberry flavour: analysis and biosynthesis. J. Sci. Food Agric. 74 (4), 421–434. doi:10.1002/(SICI)1097-0010(199708)74:4<421::AID-JSFA817>3.0.CO;2-6
Zakaria, H., Hussein, G. M., Abdel-Hadi, A.-H. A., and Abdallah, N. A. (2014). Improved regeneration and transformation protocols for three strawberry cultivars. GM Crops Food 5 (1), 27–35. doi:10.4161/gmcr.27229
Zeng, B., Li, T., Wang, W., Dai, Z., Li, J., Xi, Z., et al. (2021). An effector–reporter system to study cellular signal transduction in strawberry fruit (Fragaria ananassa). Hortic. Res. 8, 60. doi:10.1038/s41438-021-00493-3
Zhang, C., Liu, Y., Wang, B., Li, H., Zhang, J., Ma, Y., et al. (2023). CRISPR/Cas9 targeted knockout FvPHO2 can increase phosphorus content and improve fruit quality of woodland strawberry. Sci. Hortic. 317, 112078. doi:10.1016/j.scienta.2023.112078
Zhang, H., Wang, X., Pan, Q., Li, P., Liu, Y., Lu, X., et al. (2019). QTG-seq accelerates QTL fine mapping through QTL partitioning and whole-genome sequencing of bulked segregant samples. Mol. Plant 12 (3), 426–437. doi:10.1016/j.molp.2018.12.018
Zhang, J., Lei, Y., Wang, B., Li, S., Yu, S., Wang, Y., et al. (2020). The high-quality genome of diploid strawberry (Fragaria nilgerrensis) provides new insights into anthocyanin accumulation. Plant Biotechnol. J. 18 (9), 1908–1924. doi:10.1111/pbi.13351
Zhang, S., Wang, L., Zhao, R., Yu, W., Li, R., Li, Y., et al. (2018). Knockout of SlMAPK3 reduced disease resistance to Botrytis cinerea in tomato plants. J. Agric. Food Chem. 66 (34), 8949–8956. doi:10.1021/acs.jafc.8b02191
Zhang, W. W., Zhao, S. Q., Gu, S., Cao, X. Y., Zhang, Y., Niu, J. F., et al. (2022). FvWRKY48 binds to the pectate lyase FvPLA promoter to control fruit softening in Fragaria vesca. Plant Physiol. 189 (2), 1037–1049. doi:10.1093/plphys/kiac091
Zhang, Y., Iaffaldano, B., and Qi, Y. (2021a). CRISPR ribonucleoprotein-mediated genetic engineering in plants. Plant Commun. 2 (2), 100168. doi:10.1016/j.xplc.2021.100168
Zhang, Y., Ren, Q., Tang, X., Liu, S., Malzahn, A. A., Zhou, J., et al. (2021b). Expanding the scope of plant genome engineering with Cas12a orthologs and highly multiplexable editing systems. Nat. Commun. 12 (1), 1944. doi:10.1038/s41467-021-22330-w
Zhao, X., Muhammad, N., Zhao, Z., Yin, K., Liu, Z., Wang, L., et al. (2021). Molecular regulation of fruit size in horticultural plants: a review. Sci. Hortic. 288, 110353. doi:10.1016/j.scienta.2021.110353
Zhao, Y., Liu, Q., and Davis, R. E. (2004). Transgene expression in strawberries driven by a heterologous phloem-specific promoter. Plant Cell Rep. 23 (4), 224–230. doi:10.1007/s00299-004-0812-0
Zhou, J., Sittmann, J., Guo, L., Xiao, Y., Huang, X., Pulapaka, A., et al. (2021). Gibberellin and auxin signaling genes RGA1 and ARF8 repress accessory fruit initiation in diploid strawberry. Plant Physiol. 185 (3), 1059–1075. doi:10.1093/plphys/kiaa087
Zhou, J., Wang, G., and Liu, Z. (2018). Efficient genome editing of wild strawberry genes, vector development and validation. Plant Biotechnol. J. 16 (11), 1868–1877. doi:10.1111/pbi.12922
Zhou, X., Welsch, R., Yang, Y., Álvarez, D., Riediger, M., Yuan, H., et al. (2015). Arabidopsis OR proteins are the major posttranscriptional regulators of phytoene synthase in controlling carotenoid biosynthesis. Proc. Natl. Acad. Sci. U. S. A. 112 (11), 3558–3563. doi:10.1073/pnas.1420831112
Zhou, Y., Xiong, J., Shu, Z., Dong, C., Gu, T., Sun, P., et al. (2023). The telomere-to-telomere genome of Fragaria vesca reveals the genomic evolution of Fragaria and the origin of cultivated octoploid strawberry. Hortic. Res. 10 (4), uhad027. doi:10.1093/hr/uhad027
Zhu, G., Wang, S., Huang, Z., Zhang, S., Liao, Q., Zhang, C., et al. (2018). Rewiring of the fruit metabolome in tomato breeding. Cell 172 (1), 249–261. doi:10.1016/j.cell.2017.12.019
Zhu, H., Chen, M., Wen, Q., and Li, Y. (2015). Isolation and characterization of the carotenoid biosynthetic genes LCYB, LCYE and CHXB from strawberry and their relation to carotenoid accumulation. Sci. Hortic. 182, 134–144. doi:10.1016/j.scienta.2014.12.007
Zingaretti, M. L., Monfort, A., and Pérez-Enciso, M. (2019). PSBVB: a versatile simulation tool to evaluate genomic selection in polyploid species. G3 Genes, Genomes, Genet. 9 (2), 327–334. doi:10.1534/g3.118.200942
Zorrilla-Fontanesi, Y., Rambla, J.-L., Cabeza, A., Medina, J. J., Sánchez-Sevilla, J. F., Valpuesta, V., et al. (2012). Genetic analysis of strawberry fruit aroma and identification of O-methyltransferase FaOMT as the locus controlling natural variation in mesifurane content. Plant Physiol. 159 (2), 851–870. doi:10.1104/pp.111.188318
Zsögön, A., Čermák, T., Naves, E. R., Notini, M. M., Edel, K. H., Weinl, S., et al. (2018). De novo domestication of wild tomato using genome editing. Nat. Biotechnol. 36 (12), 1211–1216. doi:10.1038/nbt.4272
Keywords: CRISPR/Cas genome editing, octoploid strawberry, genome sequencing, transformation, polyploid
Citation: Vondracek K, Altpeter F, Liu T and Lee S (2024) Advances in genomics and genome editing for improving strawberry (Fragaria ×ananassa). Front. Genet. 15:1382445. doi: 10.3389/fgene.2024.1382445
Received: 05 February 2024; Accepted: 04 April 2024;
Published: 19 April 2024.
Edited by:
Rongbin Hu, University of California, Riverside, United StatesReviewed by:
Aftab Ahmad, University of Agriculture, PakistanThomas M. Davis, University of New Hampshire, United States
Copyright © 2024 Vondracek, Altpeter, Liu and Lee. This is an open-access article distributed under the terms of the Creative Commons Attribution License (CC BY). The use, distribution or reproduction in other forums is permitted, provided the original author(s) and the copyright owner(s) are credited and that the original publication in this journal is cited, in accordance with accepted academic practice. No use, distribution or reproduction is permitted which does not comply with these terms.
*Correspondence: Seonghee Lee, seonghee105@ufl.edu